Supervised by: Alexandra Knighton, BA (Oxon). Alex is a 5th year medical student at the University of Oxford who recently completed her undergraduate degree in medical sciences. Alex particularly enjoyed studying Neuroscience, with an especial interest in psychiatry, degenerative disease and circadian rhythms. She has also conducted a research project looking at ethics in global health.
Abstract
Parkinson’s disease (PD) is a neurodegenerative disease attributed to the death of dopaminergic neurons. With no cure, possible options are being developed for the future. Gene therapy is among these, which is a process that entails the genetic modification of defective or missing genetic sequences that inflict disease through the transplantation of normal genes. There is a range of different types of gene therapies currently in trial, including the use of RNAi, CRISPR, GDNF; and viral vectors such as adenoviruses, adeno-associated viruses, and lentiviruses. Gene therapy is promising for the future of PD and other neurodegenerative diseases.
Introduction
Overview of PD
PD is a progressive neurodegenerative disease categorized by four primary motor symptoms: bradykinesia, tremor rigidity, rigor, and postural instability (Kouli, Torsney and Kuan, 2018). PD has several non-motor symptoms: neuropsychiatric, autonomous, sensory symptoms, and sleep disorders (Gokcal et al., 2017). Currently, there is no definitive test for PD; this is a disease that is easily neglected or misdiagnosed (Adams, 2017). There is no cure for PD; however, there are trials that are currently trying to find potential treatments. For example, by targeting genes, developing dopamine agonists, and introducing immunity against α-synuclein (Chen and Le, 2006; Oertel and Schulz, 2016).
Epidemiology and Risk Factors
A quantitative study investigated the number of people with PD in the world’s most populated nations, estimating that by the year 2030, the number of people with PD will rise from 8.7 million to 9.3 million (Tan, 2013). PD is prevailing in older ages, affecting 1% of people over the age of 60 – this is equivalent to 127,000 people in the UK. It is prevalent in 5% of individuals over 85, proving age is a strong risk factor. (Reeve, Simcox and Turnbull, 2014; Weintraub, Comella and Horn, 2008). Other risk factors may include lifestyle and environmental factors, and having a family history of PD, tremors, constipation, anxiety, or depression (Noyce et al., 2012). Genetic mutations can also cause PD. For example, mutations in SNCA can lead to inhibited maintenance of dopaminergic neurons (Oczkowska et al., 2013), and recessive mutations in Parkin, DJ-1, and PINK1 disrupt protein coding, causing these neurons to lose certain functions (Cookson, 2010).
Pathophysiology
The main pathologic hallmark of PD relates to the degeneration of dopaminergic neurons in the substantia nigra pars compacta (SNpc) (Weintraub, Comella and Horn, 2008). The depletion of striatal dopamine is thought to be the root of motor symptoms in PD (DeMaagd and Philip, 2015). This neurodegeneration is not limited to the nigral dopaminergic neurons but also the locus coeruleus; the dorsal motor nucleus of the vagus; the nucleus basalis of Meyner; the olfactory system; and the peripheral autonomic nervous system (Kouli, Torsney and Kuan, 2018; McNaught and Olanow, 2006). Non-dopaminergic neurotransmitters affect the basal ganglia dopaminergic system. Non-dopaminergic systems that have been implicated are glutamatergic, GABAergic, cholinergic, noradrenergic, serotonergic, opioidergic, histaminergic, and adenosinergic systems (Kalia, Brotchie and Fox, 2012). According to Kouli, Torsney and Kuan (2018), degeneration in these systems may be responsible for the lack of success in treating non-motor symptoms with dopamine replacement surgeries. Due to the loss of neurons, there is a loss of neuromelanin (NM) which is indicated by the loss of brown pigment in the brain (Fasano et al., 2003).
Commonly, intracytoplasmic eosinophilic inclusions called Lewy bodies (LB) are present in surviving neurons of the substantia nigra and other areas of the brain in PD (Fahn and Sulzer, 2004). LBs are clumps of aggregated proteins, responsible for the loss of neurons (Outeiro et al., 2019; Gibb and Lees, 1988). Thus, the formation of LBs is used as an indicator of neuronal degeneration (Wakabayashi et al., 2007). Large amounts of α-synuclein are found in LB (Spillantini et al., 1998). This may suggest a disruption in proteostasis (Bentea, Verbruggen and Massie, 2017). α-Synuclein causes detrimental damage to cells nearby by causing protein aggregation, which contributes to disease propagation. By targeting the negative effects of α-synuclein with therapeutic strategies, we may come closer to the cure for PD (Stefanis, 2011).
Current Treatments
There are various approaches to treating PD. Popular treatments can be categorized into two groups: pharmacological therapies and surgical treatments. These treatments are usually symptom-alleviating, but disease-modifying treatments (curative or preventative) are currently in development.
Current pharmacological therapies only have the ability to alleviate symptoms of PD. They don’t have any neuroprotective effect and cannot alter the root of the disease – the fundamental neurodegeneration. A widely prescribed medication for PD is levodopa, which is a precursor to dopamine. It is usually combined with a peripheral decarboxylase inhibitor or carbidopa (Sancho-Bielsa, 2022). This prevents the peripheral conversion of levodopa into dopamine – which would otherwise cause adverse effects such as nausea (van Rumund et al., 2021). Currently, levodopa is the gold standard PD treatment (Leigh, 2019), due to its efficacy in managing its symptoms (especially motor), particularly during the earlier stages (Poewe et al., 2010). Nevertheless, it doesn’t work universally for everyone, having the potential to create adverse effects and lose efficacy as the disease progresses. Therefore, a new gold standard treatment for PD should be developed – a treatment universally effective that can do more than just treat motor symptoms. Gene therapy holds the potential to be this new treatment.
The effects of oral drugs such as levodopa may wear off quickly, but symptoms that appear can be offset by the administration of levodopa through a feeding tube directly to the small intestine, or through inhalation. However, the efficacy of this method greatly declines as the disease progresses as the individual could develop tolerance. Other pharmacological treatments include anticholinergic drugs, which aim to reduce symptoms of PD-caused dopaminergic neuron and nigrostriatal cell degradation; amantadine, which helps mitigate PD’s symptoms (including levodopa-induced dyskinesias); and dopamine agonists, which have high risks of adverse effects (Sancho-Bielsa, 2022).
Surgical treatments are only considered when pharmacologic treatment of PD is inadequate at suppressing an individual’s symptoms, but is restricted to reducing the severity of motor symptoms. Deep brain stimulation (DBS) is currently the preferred PD-treating surgery (Sancho-Bielsa, 2022). DBS reduces motor symptoms up to five to ten years, improves the quality of patients’ lives, and allows for the concentration and dosage of levodopa prescribed to be decreased (Hitti et al., 2019). Less common surgical treatments of PD include lesion procedures like pallidotomies, which involve destroying the pallidum in one side of the brain to decrease motor symptoms in the opposite side of the body, or sometimes even both sides of the body (Bogdanovic et al., 2016).
Overview and Mechanics of Gene Therapy
Gene therapy has the potential to be both a disease-altering and symptom-alleviating treatment. It modifies the genomes and, thereby, the functions of dysfunctional or mutated cells by replacing or adding to an absent or malfunctioning gene. This happens when a new, functional gene is inserted into the body (Sinclair, Islam and Jones, 2018) to either minimize symptoms, inhibit disease (by switching off disease-causing genes), or activate the immune response to remove diseased cells (Coune, Schneider and Aebischer, 2012; Milone and O’Doherty, 2018; Frederick, 2021; Gonçalves and Paiva, 2017). Viral vectors are a type of gene therapy that delivers genes into targeted cells by integrating their modified DNA into the DNA of the target cell through translation. Despite being derived from viruses, these are modified so as not to cause disease (Parr-Brownlie et al., 2015). However, there are hypothetical risks to this type of gene therapy, such as rejection caused by the immune system leading to inflammation and possibly organ failure, and the virus affecting the wrong cell type causing mutation in the wrong gene, resulting in damage to healthy cells and oncogenic tumor formation (Schlimgen et al., 2016).
Early clinical trials using gene therapy have shown encouraging outcomes. The development of gene therapy has been incredible so far; clinical trials are beginning to display its strong potential as a cure for PD (Mulholland, 2020).
Adenoviral and Adeno-Associated Viral Vectors
Adenoviruses and Adeno-Associated Viruses (AAVs) are viral vectors that are promising gene therapy techniques for treating PD. Adenoviruses insert a gene of interest into a eukaryotic cell where the gene is expressed (Majhen and Ambriovi-Ristov, 2006) and are replication-defective, meaning they lack one or more functionalities required for viral genome replication or viral particle production and assembly (Dudek and Knipe, 2006). These viral genes are deleted and replaced by a cassette that expresses a foreign therapeutic gene for curing the disease (Wold and Toth, 2013). Adenoviruses contain large portions of DNA (up to 8kb) that may be produced at high titers devoid of contaminating replication-competent viruses and can infect both dividing and non-dividing cells. Infecting non-diving cells is critical. The transferred DNA remains in the nucleus as a non-integrated episome, thus best suited for the transitory expression of transgenes in non-dividing cells (Björklund et al., 2000). Adenovirus vectors are effective tools for ex vivo gene therapy, involving genetically modifying cells before transplanting them into the brain system.
The efficacy of adenovirus as a gene vector system has recently been proven in numerous types of neurodegenerative illnesses, including PD. Adenoviruses expressing tyrosine hydroxylase (TH), superoxide dismutase, or glial-derived neurotrophic factor (GDNF) increased the survival and functional efficacy of dopaminergic cells in PD rat models (Barkats et al., 1998).
Another study found that intrastriatal transduction of the tyrosine hydroxylase (TH) gene can result in considerable behavioral recovery in 6-OHDA (neurotoxin 6-hydroxydopamine) lesioned rats (Horellou et al., 1994). Degeneration of dopaminergic neurons in the substantia nigra leads to a decrease in striatal dopamine levels, which is a constant neurochemical anomaly in PD. As TH catalyzes the production of levodopa, the rate-limiting stage in the manufacture of dopamine, the disease is classified as a striatal TH deficient syndrome. Thus, some animal investigations for PD use adenovirus vectors to target the TH gene (Haavik and Toska, 1998). Rats with 6-OHDA lesions of the nigrostriatal pathway are the most utilized animal model of PD. 6-OHDA is a neurotoxin that enters the brain through the dopamine-active transporter and targets catecholamine neurons. It induces widespread, irreversible death of dopamine neurons in the ventral midbrain when stereotaxically injected into the brain, either into the medial forebrain bundle (MFB) or the neostriatum. The ensuing loss of dopamine innervation in specific regions is associated with several long-term behavioral problems, which are the focus of experimental therapies aimed at preserving or restoring dopaminergic deficits. Three to four weeks after the 6-OHDA injury, an adenovirus encoding the human TH-1 cDNA under the control of the RSV promoter (Ad-RSVhTH) was stereotactically injected into nine places in the striatum. Infected striatal cells generated dopamine, reduced dopamine receptor hypersensitivity, and dramatically reduced apomorphine synthesized between 1 and 2 weeks after Ad-RSVhTH injection. Immunohistochemistry was used to detect TH production in infected tissues.
Ad-RSVhTH injection contains adenovirus vectors (Meara, 2010). Most infected cells were reactive astrocytes, but some TH-immunoreactive neurons were also seen. Reactive astrocytes alter morphology, molecular structure, and function in reaction to pathogenic circumstances in the surrounding tissue (Liddelow et al., 2017). The infection of reactive astrocytes with Ad-RSVhTH shows that astrocyte biology disturbance is involved in dopaminergic neuron loss in PD. Although the majority of PD cases are idiopathic, 17 monogenic gene mutations of astrocytes have been found and linked to the disease’s development. (Booth, Hirst and Wade-Martins, 2017). These investigations show that PD is caused, at least in part, by astrocyte dysfunction. However, infecting the reactive astrocytes reduces the mutation level and degeneration of dopaminergic neurons.
AAVs belong to the family Parvoviridae and the genus Dependoparvovirus (Cotmore et al., 2013; Hildebrandt et al., 2020). AAVs are non-pathogenic and replication-defective viruses that require adenovirus or herpes simplex virus co-infection to complete the replication cycle. AAVs can cause latent infection in the absence of a helper virus, in which the viral genome remains incorporated in infected cells (Fajardo-Serrano et al., 2021). The viral genome has been deleted from 96% of the recombinant AAV vectors, leaving only the two short inverted terminal repeats required for packaging and integration. These vectors have the benefit of being able to integrate and stably produce their transgene product in non-dividing cells, and the absence of viral genes reduces the production of foreign proteins. Thus, there is a risk of eliciting host immune responses (Björklund et al., 2000).
One study for gene transfer of dopamine-synthesizing enzymes into striatal neurons resulted in behavioral recovery in people with PD. The study looked at the safety, tolerability, and efficacy of AAV vector-mediated gene delivery of AADC into the putamen of PD patients. Six patients with a mean age of 60 (range, 51-68) years were enrolled in this study. The average disease duration was 10 years, while the time on levodopa was 9.3 years. The average daily levodopa and levodopa equivalent dosages at baseline were 642 and 808mg, respectively. As a result, intraputaminal AAV-hAADC-2 infusion significantly improved both total and motor scores of the unified PD rating scale (UPDRS) in the OFF state for five of six patients (Muramatsu et al., 2010).
In another study, transduction with AAV-TH, AAV-AADC, and AAV-GCH resulted in higher dopamine production than double transduction with AAV-TH and AAV-AADC. Additionally, triple transduction increased BH4 and dopamine synthesis in Parkinsonian rats’ denervated striatum and improved the rats’ rotating behavior more effectively than double transduction. With a stereotaxic intrastriatal injection, behavioral recovery lasted at least 12 months. These findings imply that, in addition to TH and AADC, GCH is effective in PD gene therapy (Shen et al., 2000).
Intrastriatal expression of dopamine-synthesizing enzymes TH and AADC is another promising method of PD gene therapy. AAV vectors, which are derived from non-pathogenic viruses, are the best gene-delivery vehicles for neurons. In Parkinsonian rats, TH and AADC genes were delivered into the striatum on the lesioned side using separate AAV vectors (combined with other chemicals to maximize efficacy), and coexpression of TH and AADC resulted in better behavioral recovery than TH alone. This demonstrates that AAV vectors are reliable, and can be paired with other genes to reduce motor scores (Wood et al., 1996; Kajiwara et al., 1997).
AAVs do not integrate the target cell genome since they remain episomes within the cells, but lentiviruses are integrative and offer very stable expression. AAVs are smaller particles than lentiviruses, giving them an edge in terms of spreading efficiency within certain tissues. Additionally, the expression cassette can only be 4.5kb in size, whereas a lentiviral vector can carry a 10kb insert (Duthoit, 2017). The creation of gutless adenoviral vectors enables us to avoid anti-adenoviral vector immunity. Despite concerns about their safety, there has been substantial research regarding using adenovirus vectors in a variety of therapeutic applications, and the safest dose and administration routes are now well established. Indeed, adenovirus vectors are the most commonly utilized vector in clinical studies worldwide, accounting for more than 20% of all gene therapy trials. Furthermore, adenovirus vectors provide a diverse platform for developing techniques to change viral capsids to improve therapeutic qualities and virus-targeting specificity (Lee et al., 2017). Nevertheless, the adenovirus vectors employed in GDNF transfer investigations thus far have the disadvantage of expressing adenovirus proteins, which may promote inflammation and trigger host immune responses against the infected cells (Wood et al., 1996; Kajiwara et al., 1997).
Lentiviral Vectors
Lentiviruses (LVs) are a genus of retrovirus. Retroviruses are viruses that infect other cells by inserting a DNA copy of their RNA genome into the DNA of the host cell it invades, changing the genome of that cell. The most well-known LV is the human immunodeficiency virus (HIV). Since the early 1980s, lentiviral biology has been broadly studied (Parr-Brownlie et al., 2015). If manipulated, these LVs can be used as gene therapy. Most lentiviral vectors (LVVs) produced for clinical use are derived from HIV type-1 as they maintain the ability to integrate DNA into the genome of host cells. This technology could be invaluable in both research and cures (Schlimgen et al., 2016). LVVs transfer genes effectively to normal dividing cells and non-dividing cells such as glia and neurons (Parr-Brownlie et al., 2015) to alter their function (Schlimgen et al., 2016). LVVs’ use medically has the potential to cure diseases like PD in a singular treatment as the correct and most functionally optimal genetic material is introduced and integrated into the patient’s cells (Munis, 2020).
LVVs have a variety of applications related to PD, including experimental, clinical treatments and scientific studies. The most common experimental drug utilizing LVVs is ProSavin – a type of LVV-based gene therapy that restores the production of dopamine in patients who are at a late stage of PD (Palfi et al., 2014). This works by converting non-dopaminergic cells in the striatum cells, which are not affected by PD, into dopamine-producing cells to reverse the symptoms of PD caused by the loss of dopamine (Palfi et al., 2012). Using LVVs to deliver genes directly into the striatum helps produce three enzymes: AADC, a catalyst in the conversion of levodopa into dopamine (Leveille and Blau, 2020; Christine et al., 2009); TH, an enzyme in the hydroxylation of tyrosine into levodopa (Daubner, Le and Wang, 2011); and GTP-cyclohydrolase 1, which catalyzes dopamine production in nigrostriatal cells (Mencacci et al., 2014). These genes are integrated into target cells’ genomes through LVV infection to increase dopamine production (Palfi et al., 2018). A trial testing the safety and efficacy of bilateral, intrastriatal delivery of ProSavin on a group of PD patients found that there was a remarkable improvement in the average UPDRS part III motor scores and no major adverse effects of the treatment. Six months of treatment led to a 31.6% decrease in the average UPDRS score (Palfi et al., 2014); and after two years of treatment, most patients demonstrated continuous improvement in their symptoms (Palfi et al., 2018). Overall, the treatment was well tolerated in late-stage PD patients.
Another use of LVVs was demonstrated in a study conducted by Lo Bianco et al. (2004). This was motivated by the concept that the loss of parkin’s E3-ligase activity can give rise to the degeneration of dopaminergic neurons, which leads to PD. This led researchers to believe that parkin can potentially be a key element in dopaminergic neuron survival due to its potential neuroprotective nature, and could be the key to PD prevention. This theory was evaluated by testing whether the overexpression of wild-type rat parkin could protect against the neurodegenerative effects of mutated human A30P α-synuclein in a rat lentiviral model of PD. cDNAs encoding nuclear-localized yellow fluorescent protein (YFP), A30P human α-synuclein, and rat parkin were cloned into the SIN-W-PGK lentiviral transfer vector. This produced lenti-YFP, lenti-A30P, and lenti-parkin viral particles. These LVV solutions were then injected stereotaxically into the right substantia nigra of adult female Wistar rats to infect cells and alter their genomes. Six weeks post-experiment, the rats were killed by an overdose for histological examination. Analysis via confocal microscopy found that the rats with lenti-A30P (expressing α-synuclein) experienced the death of almost all of their dopaminergic neurons within 6 weeks of the injection, whereas rats injected with both lenti-A30P and lenti-parkin had significantly less dopaminergic cell death (see Figure 1). Animals injected with lenti-parkin or lenti-YFP alone were the control groups, which had no dopaminergic neuron death, demonstrating the absence of side effects. This proves parkin can protect dopaminergic neurons against neurodegeneration (Lo Bianco et al., 2004). This technique shows that PD could be prevented or cured through the use of LVVs increasing the number of cells that secrete genes with neuroprotective qualities, such as the parkin gene. Even so, this treatment would require numerous human trials as it is unclear whether the chemicals introduced and the biological alterations would have any neurotoxic or adverse effects on people, as human and rat genomes may have dissimilarities in reaction to the treatments.
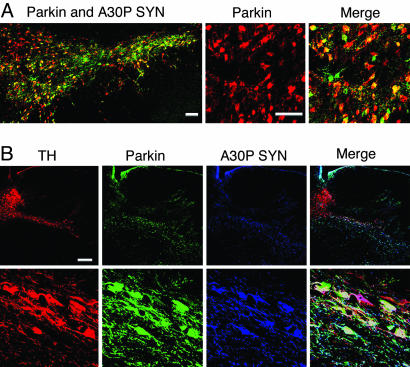
Figure 1: Confocal images of triple labeling for TH (red), parkin (green), and A30P human α-synuclein (blue) in the rat substantia nigra injected with lenti-A30P and lenti-parkin. Closer magnification (bottom row) shows the vast amount of dopaminergic neurons still secreting parkin and A30P human α-synuclein 6 weeks post lentiviral injection (Scale bars: A, 100μm; B, 250μm.) (Lo Bianco et al., 2004)
All potential PD treatments are tested on animal models, including fruit flies, rats, and non-human primate models. To reproduce dopaminergic cellular death, these models are injected with 6-hydroxydopamine and 1-methyl-4-phenyl-1,2,3,6-tetrahydropyridine. This allows for the investigation of the consequences of reduced dopamine expression, as seen in PD in humans. However, this technique does not allow for the study of the fundamental neurological pathology of PD (Sancho-Bielsa, 2022).
LVVs have many advantages. They allow for potential singular treatment cures of diseases as they can terminally transform the genome of its target cell. They also enable long-term transgene expression and gene transfer (Munis, 2020).
Despite this, there are many disadvantages. LVVs target multiple regulatory pathways and genes simultaneously, so LVV-infected cells may become cancerous through the activation of oncogenes or the silencing of tumor suppressor genes. LVVs can cause gene disruption or overexpression of normal cellular genes which could lead to infections – where the lentivirus still holds its pathogenic, replication-competent qualities, meaning it could spread to other cells and unnecessarily alter their genomes (Schlimgen et al., 2016).
Although the use of lentiviral vectors as a gene therapy is highly promising, it has yet to be released as a feasible treatment option. Tisagenlecleucel is a treatment for young people suffering from acute lymphoblastic leukemia and was approved in 2017 as the first lentiviral gene therapy to be used as a viable treatment option (Milone and O’Doherty, 2018). Nevertheless, the side effects of this treatment are severe. Many gene therapy treatments are currently in development but much more research into the long-term effects and risks needs to be conducted. Moreover, most current experimental treatments are very inconsistent and include a multitude of possible side effects of varying degrees of severity. Despite this, with years of further research and technological advancements, a range of LVV treatments for various diseases – including PD – can be developed, promising a brighter future for those affected by genetic, chronic, and/or terminal diseases (Milone and O’Doherty, 2018).
RNAi
Double-stranded RNA-mediated interference (RNAi) is a fast and simple technique for silencing gene expression, which involves the degradation of RNA into shorter RNA strands that activate ribonucleases to target homologous mRNA (Agrawal et al., 2003). Currently, scientists are silencing genes like α-synuclein as a form of gene therapy for a wide range of diseases, like PD. A widely hypothesized treatment would involve RNAi targeting the disease-causing genes. Although RNAi has only been tested in in-vitro models, it’s still a promising technology due to its ability to target specific genes, which could lead to curing disease from its root.
RNAi functions by introducing small interfering RNAs (siRNAs) into a cell in the brain that has complementary base pairing to a specific mRNA molecule. The siRNA binds to the mRNA and causes it to be degraded. When they bind, the two strands form a helical structure – lysosomes then degrade it, preventing the molecule from being translated into a protein (as ribosomes are unable to translate the double-stranded RNA). This process results in the silencing of the gene and the reduction of the proteins. This process is hypothesized to be a successful treatment, as RNAi would target only genes of interest without affecting the gene expression of others. RNAi can be administered in one of two ways: it can be delivered systemically, allowing for widespread distribution of RNAi molecules throughout the body; or it can be delivered locally to the target cells, tissues, or organs through viral vectors or nanoparticles (Xie, Woodle and Lu, 2006). The target gene and the particular RNAi therapy employed will determine the delivery strategy.
There are many advantages of RNAi. It’s considered to be a safe and suitable approach due to its low toxicity and long-lasting effects. This is due to a process called RNA-induced transcriptional silencing (RITS), which is caused by a complex protein known as the RITS complex. This contains siRNAs and is the primary mediator of RNA interference. Heterochromatin formation is associated with extensive chromatin condensation, which plays a critical role in regulating a range of chromosomal processes, including the formation of centromeres. RNAi is required for this assembly. Proper chromosome segregation and long-range chromatin interactions between distant regions are facilitated by heterochromatin. Moreover, repetitive DNA elements are known to cause genomic instability through their ability to transpose or recombine with other elements. Heterochromatin suppresses the transcription and recombination of these repetitive DNA elements (Bhattacharjee, Roche and Martienssen, 2019). RNAi has the potential to target tissues that are hard to access by other drugs. For example, the brain is difficult to access non-invasively due to the blood-brain barrier (BBB).
There are also many limitations. A challenge scientists are facing is determining how the molecules can target specific cells without triggering immune responses by the body. Additionally, the molecules could potentially bind to the wrong code of mRNA and cause the silencing of the wrong genes. This depends on the length of the RNA as shorter ones might be able to have off-target effects if the code they are sequenced against occurs naturally elsewhere. A study published in Molecular Therapy (2013) researched the use of RNAi to target ɑ-synuclein. RNAi molecules were injected directly into the brains of Parkinsonian mice and observed a reduction in the accumulation of ɑ-synuclein. However, it was noted that long-term RNAi treatment could lead to off-target effects and potential toxicity; further research is needed to determine the optimal dosage and delivery method for this type of therapy (Becker, Fischer and Grimm, 2022).
RNAi differs from other gene therapies in many ways. It can specifically target and silence the expression of particular genes, such as those involved in PD-related dopaminergic neuron degeneration, and has many ways to be administered. Some forms of gene therapy, however, might call for more invasive delivery strategies – such as injecting the gene directly into the brain or using viral vectors. The success of RNAi-based therapies will depend on several factors, including the specific RNAi therapy being used, the target gene, the method of delivery, and the specific needs of each patient. Overall, RNAi is safer compared to other gene therapy methods, making it a more desirable method to cure PD (Coune, Schneider and Aebischer, 2012).
ɑ-Synuclein and DJ-1 are among the genes that can be precisely targeted by RNAi-based treatment. Reducing the concentration of ɑ-synuclein and DJ-1 buildup may have therapeutic benefits in reducing the formation of Lewy bodies. Data collected by a group of scientists funded by the National Institute of Neurological Disorders and Stroke suggested that virally mediated RNA interference can knockdown SNCA in vivo, which could be helpful for future therapies targeting human α-synuclein (Young-Cho Kim et al., 2017). This is because the mediated knockdown of SNCA in the brains of mice led to a significant reduction in α-synuclein expression and improvements in Parkinson’s-like symptoms. A protein called DJ-1 aids in the defense of dopamine-producing neurons against oxidative stress and cellular aging. Dyskinesia, rigidity, and tremors are first observed in PD patients with a mutation in their DJ-1 protein, then further symptoms appear. By targeting negative regulators for DJ-1 expression, there would be improved survival of the DJ-1 protein, ensuring better health of the patients as these symptoms would not manifest (Oh et al., 2018).
Although RNAi-based treatments may not target symptoms specifically, they may be able to slow down the disease’s impact on the brain’s dopamine-producing neurons, helping to delay the disease’s course and lessen its symptoms.
CRISPR
CRISPR, or Clustered Regularly Interspaced Short Palindromic Repeats, is a sequence of repetitive DNA commonly referred to as CRISPR-Cas9. Cas9 is a CRISPR-associated endonuclease with a common function that acts as scissors, cutting through specific sites of DNA. CRISPR is a gene-editing tool that can repair mutated DNA by cutting specific sequences, letting DNA repair itself to sufficient health. CRISPR-Cas is a natural defense system for microbes and bacteria to recover from viruses. By creating CRISPR arrays, bacteria alter their DNA in patterns to form a CRISPR sequence. The use of CRISPR allows bacteria to build up RNA to recognize a virus and attack it if it is present again. The use of Cas9 and a similar enzyme was observed with the ability to cut the DNA and disable the virus. Thus, the direct altering of DNA seemed promising and researchers sought to adopt this process to edit human DNA. CRISPR is found at a single locus which involves two parts, clusters of genes encoding Cas proteins and CRISPR sequences. These sequences of DNA contain hundreds of repeating sequences that researchers have estimated to be 25-35 letters long (Chamary, 2022). Each DNA repeat is considered a partial palindrome, a term used in the overarching name CRISPR. A DNA palindrome is when the complementary letters of the nucleotide sequence can be read in both directions. The unique spacers found between these segments share the sequence Proto-spacer Adjacent Motif (PAM); this is the factor that allows Cas9 to be able to recognize its target (Chamry, 2022). Researchers use the concept of CRISPR to edit the genome to prevent and treat human diseases.
CRISPR is an immune defense mechanism, hence it functions differently than other gene therapy techniques. For a cell and its DNA to be modified it must produce guide RNA (gRNA). This is a mix of tracer RNA and crRNA; the produced gRNA is used to direct Cas9 to a sequence of specific genomes. Once the function of gRNA is expressed, the endonucleases of Cas9 bind to it. This complex of Cas9 can recognize the PAM (McCarty, 2020). As Cas9 binds to a PAM sequence, it checks if the gRNA and DNA strands are complementary; if they are, Cas9 performs a double-strand cut in the DNA sequence (McCarty, 2020). This cut removes potentially mutated sequences. Without the sequence, the cell repairs the gap in two ways. The first is non-homologous end joining (NHEJ), resulting in the insertion of nucleotides into the strand break. The second method is homology-directed repair (HDR), which repairs the gap through homologous recombination in the late S or G2 phase before mitosis (PubChem, 2023). If correctly performed, the use of CRISPR-Cas9 can regenerate double-stranded breaks in large amounts of cells, allowing for large quantities of genetic repair.
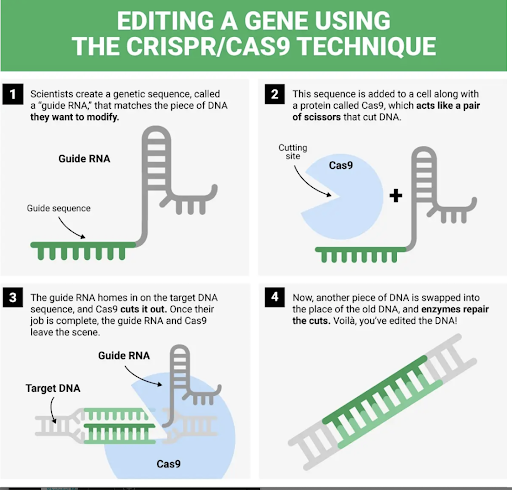
Figure 2: The steps in CRISPR-Cas9 gene therapy (Roach and Lewis, 2015).
In a laboratory, scientists must design gRNA to match the sequence of DNA intended to be edited. Different types of CRISPR tools can be more precise or general, leading to the inactivation of genes or the addition of new nucleotide sequences. Ultimately, gene editing with CRISPR is more efficient as it is customizable, leading to variety in its use, allowing for significant enhancements in gene therapy.
PD is rarely genetically inherited, though genetics plays a large part in receiving the disease and its progression. Although genetics cause 10-15% of all PD, the ability to potentially treat the uncured disease is crucial (Parkinson’s Foundation, 2017). CRISPR-Cas9 has been used to screen genetic casualties and study Parkinson’s phenotype. Neuronal cells obtain more damage with aging; this is known as the phenomenon of selective neuronal vulnerability (Biswas, 2021). Particular neuronal vulnerability is commonly reported in neurodegenerative disorders. Genetic expression has been used to prove differences between vulnerable and healthy neurons, and genetic screens are essential to understand this difference. Molecular mechanisms of PD progression are yet to be understood; though through the use of CRISPR-Cas9, we can screen the genetic variants responsible for selective neuronal vulnerability and more. A variant, CRISPRi, was tested to develop functional genetic screens for induced pluripotent stem cells (iPSC)-derived human neurons. CRISPRi has investigated genes at varying expression levels; the eventual goal is to identify potential therapeutic targets for multiple neurodegenerative conditions. The use of CRISPR screening allows for further advancements and knowledge of neurodegenerative disorders like PD. Mitophagy, redox metabolism, and aberrant mitochondria are all prominent in PD neurodegeneration. Mitochondria are key to ensuring cell health and providing energy. Cells in PD experience dysfunctional mitochondria through the process of mitophagy as a self-cleaning mechanism. These unusual mitochondrial pathways are reported in PD neurodegeneration. PINK1 and PRKN are genes that drive mitochondria functions and mitophagy and have been reported as commonly mutated in PD (Biswas, 2021). CRISPR-Cas9 is currently being used for PINK1 deletion to understand phenotypes associated with PD patients. Additionally, scientists are screening the genome with CRISPR to find the regulators of PRKN gene expression, a gene not heavily understood. With CRISPR’s promising outcomes, finding what regulates these proteins is more efficient. CRISPR is being used as a spectrum to treat PD, driving various genes and methods like screening and specifically targeting genes to find a cure – hence it is a promising technique.
GDNF
One protein that could be delivered using the above techniques is GDNF. The glial cell line-derived neurotrophic factor (GDNF) is a neurotrophic factor that can be used therapeutically to target dopaminergic neurons. During PD, dopaminergic neurons tend to degenerate, and this agent aims to prevent that from happening (Hunot et al., 1996). In literature, GDNF has proven to be a successful tool that allows for long-term motoneuron survival (Eggers et al., 2020). Though the induction of GDNF is short, its effects seem to last longer (Tereshchenko et al., 2014).
GDNF is a protein that cannot pass the BBB. However, GDNF could be altered to aid gene therapy through the delivery of viral vectors. GDNF has achieved positive results with the help of AAVs. A recent study regarding an AAV α-synuclein model showed that the GDNF’s inability to perform well in humans may be due to α-synuclein overexpressing nigral neurons to meet GDNF’s demand. The study suggests that this may be due to the down-regulation of the receptor RET caused by a decrease in the protein Nurr1, which is in charge of regulating RET expression in dopaminergic neurons (Barker et al., 2020). This is further backed up by an experiment involving Nurr1-depleted mice, which proved that GDNF is ineffective in dopaminergic neurons without Nurr1. The RET expressions decreased as there was no Nurr1 present that inhibited response to GDNF; when Nurr1 was restored, dopaminergic neurons survived against α-synuclein (Decressac et al., 2012).
In the trial done by Choi-Lundberg et al. (1997), a replication-defective adenovirus encoding GDNF (AD-GDNF) was injected near the substantia nigra. 6-OHDA was also injected into the striatum. AD-GDNF successfully protected dopaminergic neurons from degeneration caused by 6-OHDA. Effects lasted for 6 weeks, proving the benefits of GDNF as it can have long-lasting effects, further insinuating that AD-GDNF gene therapy may be the future of slowing down cell loss in PD. Similarly, a trial done by Kordower (2000) focused on using lentiviral vectors to deliver GDNF to the substantia nigra and the striatum. The study proved that the use of LVVs to aid GDNF delivery would prevent nigrostriatal degeneration, demonstrating that this may be a possible therapeutic option for PD patients. It also showed that the effects of GDNF were long-lasting, proving its potential once again. However, as this study was on non-lesioned and young adult rhesus monkeys, rather than older monkeys, there was no way to measure the effectiveness of this method on later stages of PD compared to earlier stages of PD.
Recently there have also been attempts at non-viral GDNF gene therapy. The new intravenous GDNF gene therapy involves monoclonal antibodies (MAb) targeting Trojan horse liposomes (THLs ) to the rat transferrin receptor (TfR). Previously, similar trials were conducted, but Zhang and Pardridge (2008) aimed to focus on the effect of using THL gene therapy for a longer time. The results were positive; almost perfect removal of neurotoxin effects was achieved. Given the results, we can see that further development of non-viral GDNF gene therapy may be useful in the future. As this trial was on mice, it would be significant if similar results were achieved in a human trial.
Regarding the benefits, several clinical trials on the protein prove the significance of this protein in aiding neuron protection (Barker et al., 2020; Bartus et al., 2013). Early on, trials on Transgenic SOD1 mice found that GDNF could demonstrate neuroprotective effects (Acsadi et al., 2002). A double-blind trial where human subjects were given placebos of GDNF was also conducted; this was done via implanting an intracerebroventricular catheter. The trial failed as it was unable to meet the targeted areas, which were the putamen and the substantia nigra (Nutt et al., 2003). This was because GDNF, being a protein, was unable to pass the BBB, and there were brain diffusion and pharmacodynamic issues (Lindvall and Wahlberg, 2008). Another trial focused on infusing the protein I125-GDNF into the putamen of three adult rhesus monkeys. After seven days, the infused GDNF had uneven distributions, especially around the catheter. This showed that there was limited diffusion of GDNF into the brain parenchyma (Salvatore et al., 2006).
Although GDNF has proven to be safe, one of the members from the same family, Neuturin (structurally similar to GDNF), has shown data that suggests otherwise (Manfredsson et al., 2020; Barker et al., 2020). A study conducted by Marks Jr et al. (2010) involved the gene delivery of Neurturin via an AAV type 2. The trial was a double-blind sham surgery, aiming to evaluate the safety of the gene. Patients of PD were randomly assigned to receive either the AAV-Neurturin or the sham surgery. There were no significant results but rather, there were many side effects post-experiment. Thus, this exhibited the potential dangers of GDNF.
In conclusion, GDNF and its variants definitely have a place in gene therapy in the future. As seen with the viral and non-viral GDNF gene therapies, the results have been positive. Therefore, GDNF has a promising place in the future of gene therapy and finding a treatment for PD. The animal clinical trial studies are strong, but there is not enough evidence supported by human clinical trials. Therefore, more research and clinical trials are required to ensure the safety of the strategy.
The Use of Viruses to Cross the Blood-Brain Barrier
The blood-brain barrier (BBB) consists of tight junctions and highly specialized endothelial cells (Norsted, Gömüç and Meister, 2008; Lee, 2014). Being part of one of the immunological features of the central nervous system, it is semi-permeable; 98% of small molecules and almost all large molecules are unable to pass through to the brain. Unfortunately, this defense system prevents anti-PD drugs from passing the membrane (Cheng et al., 2022).
An approach to transporting viral vectors across the BBB would be via temporary disruption of brain microvascular endothelial tight junctions (Fu and McCarty, 2016). For example, the use of lentiviruses. As the LDLR (low-density lipoprotein receptor) can find apolipoproteins at the BBB, this would allow it to transcytose to the abluminal side of the BBB. Utilizing this information, a team tested if a recombinant protein can bind to the LDLR to transcytose into the central nervous system (CNS). The study found out that the LDLR-binding domain of ApoB was able to produce a secreted form of GFP or the secreted form of glucocerebrosidase on the other side, demonstrating that the use of lentiviral vectors in crossing the BBB may show promise in carrying proteins and genes to the CNS, aiding in gene therapy (Spencer and Verma, 2007).
Furthermore, there has been use of RNAi in crossing the BBB. Usually, siRNA and viral vectors are directly injected into the brain in gene therapy experiments. This has proven to be problematic as these methods only work in delivery around the injection site, but not throughout the whole brain. It is also too invasive. However, it is believed that less invasive methods may be developed through the use of RNAi. A study using rabies virus glycoprotein-derived peptides to deliver RNAi through the BBB showed that the delivery and transduction of neurons were feasible (Kumar et al., 2007). Hence, this shows that RNAi may be successful in crossing the BBB and becoming the future of gene therapy.
Transient disruption of tight junctions can be done with Ca2+ chelator agents, non-ionic detergents, and antibodies. In literature, it is often stated that antibodies that are re-engineered and directed to recognize endogenous transport systems reach the brain (Neves et al., 2016; Banks et al., 2002; Friden, 1997). Similar to PD, a study directed toward Alzheimer’s disease investigated the ability of AβP targeted antibodies in crossing the BBB in mice. Although it was at a slow rate, the study proved that antibodies could pass the BBB when customized. In conclusion, there is promise for this method of drug delivery in PD in the future (Banks et al., 2002).
Thus, by developing these methods they could inhibit proteins from participating in tight junction complexes, which would increase permeability, allowing some viruses to pass (Ferrari, Geddes and Alton, 2002). An alternative would be through the use of receptor-mediated transcytosis, which involves the transport of a substance through brain endothelial cells toward the brain parenchyma (Fu and McCarty, 2016; Tang et al., 2006).
Conclusion
Gene therapy offers a promising future for individuals suffering from PD, having a range of potential treatments that aim to alter the genomes and natures of cells. The future of CRISPR is especially promising. Scientists are taking initiative to further research. For example, the Alliance for Therapies in Neuroscience has been recently formed, which is aiming to study and produce information about the pathophysiology of PD and its potential treatments (Biswas, 2021). Overall, the future of PD lies within the research and effort put forth to advance scientific knowledge, to come closer to a cure. Gene therapy is undoubtedly the next step in this advancement.
References
Adams, W.R. (2017). High-accuracy Detection of Early Parkinson’s Disease Using Multiple Characteristics of Finger Movement While Typing. PLOS ONE, 12(11), p.e0188226. doi:https://doi.org/10.1371/journal.pone.0188226.
Agrawal, N., Dasaradhi, P.V.N., Mohmmed, A., Malhotra, P., Bhatnagar, R.K. and Mukherjee, S.K. (2003a). RNA Interference: Biology, Mechanism, and Applications. Microbiology and Molecular Biology Reviews, [online] 67(4), pp.657–685. doi:https://doi.org/10.1128/mmbr.67.4.657-685.2003.
Agrawal, N., Dasaradhi, P.V.N., Mohmmed, A., Malhotra, P., Bhatnagar, R.K. and Mukherjee, S.K. (2003b). RNA Interference: Biology, Mechanism, and Applications. Microbiology and Molecular Biology Reviews, [online] 67(4), pp.657–685. doi:https://doi.org/10.1128/mmbr.67.4.657-685.2003.
Axelsen, T.M. and Woldbye, D.P.D. (2018). Gene Therapy for Parkinson’s Disease, An Update. Journal of Parkinson’s Disease, 8(2), pp.195–215. doi:https://doi.org/10.3233/jpd-181331.
Balazs, D.A. and Godbey, WT. (2011). Liposomes for Use in Gene Delivery. Journal of Drug Delivery, [online] 2011(PMC3066571), pp.1–12. doi:https://doi.org/10.1155/2011/326497.
Banks, W.A., Terrell, B., Farr, S.A., Robinson, S.M., Nonaka, N. and Morley, J.E. (2002). Passage of Amyloid Β Protein Antibody across the Blood–brain Barrier in a Mouse Model of Alzheimer’s Disease. Peptides, 23(12), pp.2223–2226. doi:https://doi.org/10.1016/s0196-9781(02)00261-9.
Barkats, M., Bilang-Bleuel, A., Buc-Caron, M.H., Castel-Barthe, M.N., Corti, O., Finiels, F., Horellou, P., Revah, F., Sabate, O. and Mallet, J. (1998). Adenovirus in the brain: Recent Advances of Gene Therapy for Neurodegenerative Diseases. Progress in Neurobiology, 55(4), pp.333–341. doi:https://doi.org/10.1016/s0301-0082(98)00028-8.
Barker, R.A., Björklund, A., Gash, D.M., Whone, A., Van Laar, A., Kordower, J.H., Bankiewicz, K., Kieburtz, K., Saarma, M., Booms, S., Huttunen, H.J., Kells, A.P., Fiandaca, M.S., Stoessl, A.J., Eidelberg, D., Federoff, H., Voutilainen, M.H., Dexter, D.T., Eberling, J. and Brundin, P. (2020). GDNF and Parkinson’s Disease: Where Next? A Summary from a Recent Workshop. Journal of Parkinson’s Disease, [online] 10(3), pp.875–891. doi:https://doi.org/10.3233/JPD-202004.
Bartus, R.T., Baumann, T.L., Brown, L., Kruegel, B.R., Ostrove, J.M. and Herzog, C.D. (2013). Advancing Neurotrophic Factors as Treatments for age-related Neurodegenerative diseases: Developing and Demonstrating ‘clinical proof-of-concept’ for AAV-neurturin (CERE-120) in Parkinson’s Disease. Neurobiology of Aging, 34(1), pp.35–61. doi:https://doi.org/10.1016/j.neurobiolaging.2012.07.018.
Becker, J., Fischer, N. and Grimm, D. (2022). Right on target: the next Class of efficient, safe, and Specific RNAi Triggers. Molecular Therapy – Nucleic Acids, [online] 28(P363-365), pp.363–365. doi:https://doi.org/10.1016/j.omtn.2022.03.027.
Bentea, E., Verbruggen, L. and Massie, A. (2017). The Proteasome Inhibition Model of Parkinson’s Disease. Journal of Parkinson’s Disease, 7(1), pp.31–63. doi:https://doi.org/10.3233/jpd-160921.
Bhattacharjee, S., Roche, B. and Martienssen, R.A. (2019). RNA-induced Initiation of Transcriptional Silencing (RITS) Complex Structure and Function. RNA Biology, 16(9), pp.1133–1146. doi:https://doi.org/10.1080/15476286.2019.1621624.
Biswas, T. (2021). Synthego | Full Stack Genome Engineering. [online] Available at: https://www.synthego.com/blog/crispr-parkinson-research.
Björklund, A., Kirik, D., Rosenblad, C., Georgievska, B., Lundberg, C. and Mandel, R.J. (2000). Towards a Neuroprotective Gene Therapy for Parkinson’s disease: Use of adenovirus, AAV and Lentivirus Vectors for Gene Transfer of GDNF to the Nigrostriatal System in the Rat Parkinson Model. Brain Research, [online] 886(1-2), pp.82–98. doi:https://doi.org/10.1016/s0006-8993(00)02915-2.
Bogdanovic, M., Forrow, B., Rebelo, P. and Fletcher, C. (2016). Thalamotomy and Pallidotomy Pre-operative Information for People with Tremor and/or Parkinson’s Disease. [online] Available at: https://www.ouh.nhs.uk/patient-guide/leaflets/files/10116Pthalamotomy.pdf [Accessed 20 Feb. 2023].
Booth, H.D.E., Hirst, W.D. and Wade-Martins, R. (2017). The Role of Astrocyte Dysfunction in Parkinson’s Disease Pathogenesis. Trends in Neurosciences, [online] 40(6), pp.358–370. doi:https://doi.org/10.1016/j.tins.2017.04.001.
Campbell, M., Kiang, A.-S., Kenna, P.F., Kerskens, C., Blau, C., O’Dwyer, L., Tivnan, A., Kelly, J.A., Brankin, B., Farrar, G.-J. and Humphries, P. (2008). RNAi-mediated Reversible Opening of the blood-brain Barrier. The Journal of Gene Medicine, 10(8), pp.930–947. doi:https://doi.org/10.1002/jgm.1211.
Center for Biologics Evaluation and Research (2020). What is Gene Therapy? FDA. [online] Available at: https://www.fda.gov/vaccines-blood-biologics/cellular-gene-therapy-products/what-gene-therapy#:~:text=Gene%20therapy%20is%20a%20technique [Accessed 25 Feb. 2023].
Chamary, J. (2022). CRISPR Explained – Novatein Biosciences. [online] novateinbio.com. Available at: https://novateinbio.com/content/96-crispr-explained.
Chen, S. and Le, W. (2006). Neuroprotective Therapy in Parkinson Disease. American Journal of Therapeutics, 13(5), pp.445–457. doi:https://doi.org/10.1097/01.mjt.0000174353.28012.a7.
Cheng, G., Liu, Y., Ma, R., Cheng, G., Guan, Y., Chen, X., Wu, Z. and Chen, T. (2022). Anti-Parkinsonian Therapy: Strategies for Crossing the Blood–Brain Barrier and Nano-Biological Effects of Nanomaterials. Nano-Micro Letters, 14(1). doi:https://doi.org/10.1007/s40820-022-00847-z.
Choi-Lundberg, D.L. (1997). Dopaminergic Neurons Protected from Degeneration by GDNF Gene Therapy. Science, 275(5301), pp.838–841. doi:https://doi.org/10.1126/science.275.5301.838.
Christine, C.W., Starr, P.A., Larson, P.S., Eberling, J.L., Jagust, W.J., Hawkins, R.A., VanBrocklin, H.F., Wright, J.F., Bankiewicz, K.S. and Aminoff, M.J. (2009). Safety and tolerability of putaminal AADC gene therapy for Parkinson disease. Neurology, 73(20), pp.1662–1669. doi:https://doi.org/10.1212/wnl.0b013e3181c29356.
Cookson, M.R. (2010). Chapter 3 – Unravelling the role of defective genes. [online] ScienceDirect. Available at: https://www.sciencedirect.com/science/article/abs/pii/S0079612310830031 [Accessed 24 Feb. 2023].
Cotmore, S.F., Agbandje-McKenna, M., Chiorini, J.A., Mukha, D.V., Pintel, D.J., Qiu, J., Soderlund-Venermo, M., Tattersall, P., Tijssen, P., Gatherer, D. and Davison, A.J. (2013). The family Parvoviridae. Archives of Virology, [online] 159(5), pp.1239–1247. doi:https://doi.org/10.1007/s00705-013-1914-1.
Coune, P.G., Schneider, B.L. and Aebischer, P. (2012). Parkinson’s Disease: Gene Therapies. Cold Spring Harbor Perspectives in Medicine, [online] 2(4), pp.a009431–a009431. doi:https://doi.org/10.1101/cshperspect.a009431.
Daubner, S.C., Le, T. and Wang, S. (2011). Tyrosine hydroxylase and regulation of dopamine synthesis. Archives of Biochemistry and Biophysics, [online] 508(1), pp.1–12. doi:https://doi.org/10.1016/j.abb.2010.12.017.
Decressac, M., Kadkhodaei, B., Mattsson, B., Laguna, A., Perlmann, T. and Bjorklund, A. (2012). -Synuclein-Induced Down-Regulation of Nurr1 Disrupts GDNF Signaling in Nigral Dopamine Neurons. Science Translational Medicine, 4(163), pp.163ra156–163ra156. doi:https://doi.org/10.1126/scitranslmed.3004676.
DeMaagd, G. and Philip, A. (2015). Parkinson’s Disease and Its Management: Part 1: Disease Entity, Risk Factors, Pathophysiology, Clinical Presentation, and Diagnosis. P & T : a peer-reviewed journal for formulary management, [online] 40(8), pp.504–32. Available at: https://www.ncbi.nlm.nih.gov/pmc/articles/PMC4517533/#:~:text=Striatal%20dopamine%20depletion%20has%20been [Accessed 14 Feb. 2023].
Dudek, T. and Knipe, D.M. (2006). Replication-defective viruses as vaccines and vaccine vectors. Virology, 344(1), pp.230–239. doi:https://doi.org/10.1016/j.virol.2005.09.020.
Duthoit, C. (2017). AAV or lentiviral vectors? [online] Flash Therapeutics. Available at: https://www.flashtherapeutics.com/flash-academy/blog/aav-or-lentiviral-vectors-what-should-i-use-for-my-in-vivo-experiments-106#:~:text=AAVs%20are%20smaller%20particles%20than [Accessed 25 Feb. 2023].
Eggers, R., Winter, F.D., Tannemaat, M.R., Malessy, M.J.A. and Verhaagen, J. (2020). GDNF Gene Therapy to Repair the Injured Peripheral Nerve. [online] GDNF Gene Therapy to Repair the Injured Peripheral Nerve. Available at: https://www.frontiersin.org/articles/10.3389/fbioe.2020.583184/full [Accessed 24 Feb. 2023].
Fahn, S. and Sulzer, D. (2004). Neurodegeneration and Neuroprotection in Parkinson Disease. NeuroRx, [online] 1(1), pp.139–154. Available at: https://www.ncbi.nlm.nih.gov/pmc/articles/PMC534919/#:~:text=PD%20and%20the%20Parkinson%2Dplus.
Fajardo-Serrano, A., Rico, A.J., Roda, E., Honrubia, A., Arrieta, S., Ariznabarreta, G., Chocarro, J., Lorenzo-Ramos, E., Pejenaute, A., Vázquez, A. and Lanciego, J.L. (2021). Adeno-Associated Viral Vectors as Versatile Tools for Parkinson’s Research, Both for Disease Modeling Purposes and for Therapeutic Uses. International Journal of Molecular Sciences, [online] 22(12), p.6389. doi:https://doi.org/10.3390/ijms22126389.
Fasano, M., Giraudo, S., Coha, S., Bergamasco, B. and Lopiano, L. (2003). Residual substantia nigra neuromelanin in Parkinson’s disease is cross-linked to α-synuclein. Neurochemistry International, 42(7), pp.603–606. doi:https://doi.org/10.1016/s0197-0186(02)00161-4.
Ferrari, S., Geddes, D.M. and Alton, E.W.F.W. (2002). Barriers to and new approaches for gene therapy and gene delivery in cystic fibrosis. Advanced Drug Delivery Reviews, 54(11), pp.1373–1393. doi:https://doi.org/10.1016/s0169-409x(02)00145-x.
Fire, A. and Mellow, C. (2016). The Nobel Prize in Physiology or Medicine 2006. [online] NobelPrize.org. Available at: https://www.nobelprize.org/prizes/medicine/2006/7474-the-nobel-prize-in-physiology-or-medicine-2006-2006-4/.
Frederick, E. (2021). An on-off switch for gene editing. [online] MIT News | Massachusetts Institute of Technology. Available at: https://news.mit.edu/2021/switch-crispr-gene-editing-0414.
Friden, P.M. (1997). Utilization of an endogenous cellular transport system for the delivery of therapeutics across the blood–brain barrier. Journal of Controlled Release, 46(1-2), pp.117–128. doi:https://doi.org/10.1016/s0168-3659(96)01580-5.
Fu, H. and McCarty, D.M. (2016). Crossing the blood–brain-barrier with viral vectors. Current Opinion in Virology, 21, pp.87–92. doi:https://doi.org/10.1016/j.coviro.2016.08.006.
Gibb, W.R. and Lees, A.J. (1988). The relevance of the Lewy body to the pathogenesis of idiopathic Parkinson’s disease. Journal of Neurology, Neurosurgery & Psychiatry, [online] 51(6), pp.745–752. doi:https://doi.org/10.1136/jnnp.51.6.745.
Gokcal, E., Gur, V.E., Selvitop, R., Babacan Yildiz, G. and Asil, T. (2017). Motor and Non-Motor Symptoms in Parkinson’s Disease: Effects on Quality of Life. Noro Psikiyatri Arsivi, 54(2), pp.143–148. doi:https://doi.org/10.5152/npa.2016.12758.
Gonçalves, G.A.R. and Paiva, R. de M.A. (2017). Gene therapy: advances, challenges and perspectives. Einstein (São Paulo), [online] 15(3), pp.369–375. doi:https://doi.org/10.1590/s1679-45082017rb4024.
Graham, F.L. (2000). Adenovirus vectors for high-efficiency gene transfer into mammalian cells. Immunology Today, 21(9), pp.426–428. doi:https://doi.org/10.1016/s0167-5699(00)01676-5.
Haavik, J. and Toska, K. (1998). Tyrosine hydroxylase and Parkinson’s disease. Molecular Neurobiology, [online] 16(3), pp.285–309. doi:https://doi.org/10.1007/bf02741387.
Hildebrandt, E., Penzes, J.J., Gifford, R.J., Agbandje-Mckenna, M. and Kotin, R.M. (2020). Evolution of dependoparvoviruses across geological timescales—implications for design of AAV-based gene therapy vectors. Virus Evolution, 6(2). doi:https://doi.org/10.1093/ve/veaa043.
Hitti, F.L., Yang, A.I., Gonzalez-Alegre, P. and Baltuch, G.H. (2019). Human gene therapy approaches for the treatment of Parkinson’s disease: An overview of current and completed clinical trials. Parkinsonism & Related Disorders, [online] 66(1353-8020), pp.16–24. doi:https://doi.org/10.1016/j.parkreldis.2019.07.018.
Horellou, P., Brundin, P., Kalén, P., Mallet, J. and Björklund, A. (1990). In vivo release of DOPA and dopamine from genetically engineered cells grafted to the denervated rat striatum. Neuron, [online] 5(4), pp.393–402. doi:https://doi.org/10.1016/0896-6273(90)90078-T.
Horellou, P., Vigne, E., Castel, M.-N., Barnéoud, P., Colin, P., Perricaudet, M., Delaère, P. and Mallet, J. (1994). Direct intracerebral gene transfer of an adenoviral vector expressing tyrosine hydroxylase in a rat model of Parkinson’s disease. NeuroReport, [online] 6(1), p.49. Available at: https://journals.lww.com/neuroreport/Abstract/1994/12300/Direct_intracerebral_gene_transfer_of_an.14.aspx [Accessed 22 Feb. 2023].
Hunot, S., Bernard, V., Faucheux, B., Boissière, F., Leguern, E., Brana, C., Gautris, P.P., Guérin, J., Bloch, B., Agid, Y. and Hirsch, E.C. (1996). Glial cell line-derived neurotrophic factor (GDNF) gene expression in the human brain: A post mortem in situ hybridization study with special reference to Parkinson’s disease. Journal of Neural Transmission, 103(8-9), pp.1043–1052. doi:https://doi.org/10.1007/bf01291789.
John Hopkins Medicine (2019). Parkinson’s Disease and Dementia. [online] John Hopkins Medicine. Available at: https://www.hopkinsmedicine.org/health/conditions-and-diseases/parkinsons-disease/parkinsons-disease-and-dementia.
Kajiwara, K., Byrnes, A.P., Charlton, H.M., Wood, M.J.A. and Wood, K.J. (1997). Immune Responses to Adenoviral Vectors during Gene Transfer in the Brain. Human Gene Therapy, 8(3), pp.253–265. doi:https://doi.org/10.1089/hum.1997.8.3-253.
Kalia, L.V., Brotchie, J.M. and Fox, S.H. (2012). Novel nondopaminergic targets for motor features of Parkinson’s disease: Review of recent trials. Movement Disorders, 28(2), pp.131–144. doi:https://doi.org/10.1002/mds.25273.
Khanal, S., Ghimire, P. and Dhamoon, A. (2018). The Repertoire of Adenovirus in Human Disease: The Innocuous to the Deadly. Biomedicines, 6(1), p.30. doi:https://doi.org/10.3390/biomedicines6010030.
Kordower, J.H. (2000). Neurodegeneration Prevented by Lentiviral Vector Delivery of GDNF in Primate Models of Parkinson’s Disease. Science, 290(5492), pp.767–773. doi:https://doi.org/10.1126/science.290.5492.767.
Kouli, A., Torsney, K.M. and Kuan, W.-L. (2018). Parkinson’s Disease: Etiology, Neuropathology, and Pathogenesis. Parkinson’s Disease: Pathogenesis and Clinical Aspects, [online] pp.3–26. doi:https://doi.org/10.15586/codonpublications.parkinsonsdisease.2018.ch1.
Kumar, P., Wu, H., McBride, J.L., Jung, K.-E., Kim, M.H., Davidson, B.L., Lee, S.K., Shankar, P. and Manjunath, N. (2007). Transvascular delivery of small interfering RNA to the central nervous system. Nature, [online] 448(7149), pp.39–43. doi:https://doi.org/10.1038/nature05901.
Lang, A.E., Gill, S., Patel, N.K., Lozano, A., Nutt, J.G., Penn, R., Brooks, D.J., Hotton, G., Moro, E., Heywood, P., Brodsky, M.A., Burchiel, K., Kelly, P., Dalvi, A., Scott, B., Stacy, M., Turner, D., Wooten, V.G.F., Elias, W.J. and Laws, E.R. (2006). Randomized controlled trial of intraputamenal glial cell line-derived neurotrophic factor infusion in Parkinson disease. Annals of Neurology, [online] 59(3), pp.459–466. doi:https://doi.org/10.1002/ana.20737.
Lee, C.S., Bishop, E.S., Zhang, R., Yu, X., Farina, E.M., Yan, S., Zhao, C., Zeng, Z., Shu, Y., Wu, X., Lei, J., Li, Y., Zhang, W., Yang, C., Wu, K., Wu, Y., Ho, S., Athiviraham, A., Lee, M.J. and Wolf, J.M. (2017). Adenovirus-mediated gene delivery: Potential applications for gene and cell-based therapies in the new era of personalized medicine. Genes & Diseases, [online] 4(2), pp.43–63. doi:https://doi.org/10.1016/j.gendis.2017.04.001.
Lee, H. (2014). Disruption of the blood-brain barrier in parkinson’s disease: curse or route to a cure? Frontiers in Bioscience, 19(2), p.272. doi:https://doi.org/10.2741/4206.
Leigh, S. (2019). Gene Therapy Shows Initial Promise for Parkinson’s Disease. [online] Gene Therapy Shows Initial Promise for Parkinson’s Disease | UC San Francisco. Available at: https://www.ucsf.edu/news/2019/03/413696/gene-therapy-shows-initial-promise-parkinsons-disease [Accessed 15 Feb. 2023].
Leveille, E. and Blau, N. (2020). Aromatic L-Amino Acid Decarboxylase Deficiency. [online] NORD (National Organization for Rare Disorders). Available at: https://rarediseases.org/rare-diseases/aromatic-l-amino-acid-decarboxylase-deficiency/ [Accessed 24 Feb. 2023].
Liddelow, S.A., Guttenplan, K.A., Clarke, L.E., Bennett, F.C., Bohlen, C.J., Schirmer, L., Bennett, M.L., Münch, A.E., Chung, W.-S., Peterson, T.C., Wilton, D.K., Frouin, A., Napier, B.A., Panicker, N., Kumar, M., Buckwalter, M.S., Rowitch, D.H., Dawson, V.L., Dawson, T.M. and Stevens, B. (2017). Neurotoxic reactive astrocytes are induced by activated microglia. Nature, 541(7638), pp.481–487. doi:https://doi.org/10.1038/nature21029.
Lindvall, O. and Wahlberg, L.U. (2008). Encapsulated cell biodelivery of GDNF: A novel clinical strategy for neuroprotection and neuroregeneration in Parkinson’s disease? Experimental Neurology, [online] 209(1), pp.82–88. doi:https://doi.org/10.1016/j.expneurol.2007.08.019.
Liu, Y., Wang, S., Luo, S., Li, Z., Liang, F., Zhu, Y., Pei, Z. and Huang, R. (2016). Intravenous PEP-1-GDNF is protective after focal cerebral ischemia in rats. Neuroscience Letters, [online] 617, pp.150–155. doi:https://doi.org/10.1016/j.neulet.2016.02.017.
Lo Bianco, C., Schneider, B.L., Bauer, M., Sajadi, A., Brice, A., Iwatsubo, T. and Aebischer, P. (2004). Lentiviral Vector Delivery of Parkin Prevents Dopaminergic Degeneration in an α-synuclein Rat Model of Parkinson’s Disease. Proceedings of the National Academy of Sciences of the United States of America, [online] 101(50), pp.17510–17515. doi:https://doi.org/10.1073/pnas.0405313101.
Lundberg, C., Horellou, P., Mallet, J. and Björklund, A. (1996). Generation of DOPA-Producing Astrocytes by Retroviral Transduction of the Human Tyrosine Hydroxylase Gene: In-Vitro Characterization and In-Vivo Effects in the Rat Parkinson Model. Experimental Neurology, [online] 139(1), pp.39–53. doi:https://doi.org/10.1006/exnr.1996.0079.
Maheu, M., Lopez, J.P., Crapper, L., Davoli, M.A., Turecki, G. and Mechawar, N. (2015). MicroRNA regulation of central glial cell line-derived neurotrophic factor (GDNF) signalling in depression. Translational Psychiatry, 5(2), pp.e511–e511. doi:https://doi.org/10.1038/tp.2015.11.
Majhen, D. and Ambriović-Ristov, A. (2006). Adenoviral vectors—How to use them in cancer gene therapy? Virus Research, 119(2), pp.121–133. doi:https://doi.org/10.1016/j.virusres.2006.02.001.
Manfredsson, F.P., Polinski, N.K., Subramanian, T., Boulis, N., Wakeman, D.R. and Mandel, R.J. (2020). The Future of GDNF in Parkinson’s Disease. Frontiers in Aging Neuroscience, 12. doi:https://doi.org/10.3389/fnagi.2020.593572.
Market Data Forecast Ltd. (2022). RNAi Drug Delivery Market Size, Trends, Growth | 2022 to 2027. [online] Market Data Forecast. Available at: https://www.marketdataforecast.com/market-reports/global-rnai-drug-delivery-market [Accessed 22 Feb. 2023].
Marks Jr, W.J., Bartus, R.T., Siffert, J., Davis, C.S., Lozano, A., Boulis, N., Vitek, J., Stacy, M., Turner, D., Verhagen, L., Bakay, R., Watts, R., Guthrie, B., Jankovic, J., Simpson, R., Tagliati, M., Alterman, R., Stern, M., Baltuch, G. and Starr, P.A. (2010). Gene delivery of AAV2-neurturin for Parkinson’s disease: a double-blind, randomised, controlled trial. The Lancet Neurology, [online] 9(12), pp.1164–1172. doi:https://doi.org/10.1016/S1474-4422(10)70254-4.
Marras, C., Beck, J.C., Bower, J.H., Roberts, E., Ritz, B., Ross, G.W., Abbott, R.D., Savica, R., Van Den Eeden, S.K., Willis, A.W. and Tanner, C. (2018). Prevalence of Parkinson’s disease across North America. npj Parkinson’s Disease, [online] 4(1). doi:https://doi.org/10.1038/s41531-018-0058-0.
Mayo Clinic (2022). Parkinson’s disease – Diagnosis and treatment – Mayo Clinic. [online] www.mayoclinic.org. Available at: https://www.mayoclinic.org/diseases-conditions/parkinsons-disease/diagnosis-treatment/drc-20376062#:~:text=(Rytary%2C%20Sinemet%2C%20Duopa%2C [Accessed 15 Feb. 2023].
McCarty, N. (2020). CRISPR — How It Works, Top Applications and How to Use It Yourself. [online] Medium. Available at: https://nikomccarty.medium.com/almost-everything-you-should-know-about-crispr-how-it-works-top-applications-and-how-to-use-it-61e27b04bea6.
McNaught, K.St.P. and Olanow, C.W. (2006). Protein aggregation in the pathogenesis of familial and sporadic Parkinson’s disease. Neurobiology of Aging, 27(4), pp.530–545. doi:https://doi.org/10.1016/j.neurobiolaging.2005.08.012.
Meara, J. (2010). CHAPTER 64 – Parkinsonism and Other Movement Disorders. [online] ScienceDirect. Available at: https://www.sciencedirect.com/science/article/pii/B9781416062318100649 [Accessed 25 Feb. 2023].
Mencacci, N.E., Isaias, I.U., Reich, M.M., Ganos, C., Plagnol, V., Polke, J.M., Bras, J., Hersheson, J., Stamelou, M., Pittman, A.M., Noyce, A.J., Mok, K.Y., Opladen, T., Kunstmann, E., Hodecker, S., Münchau, A., Volkmann, J., Samnick, S., Sidle, K. and Nanji, T. (2014). Parkinson’s disease in GTP cyclohydrolase 1 mutation carriers. Brain, 137(9), pp.2480–2492. doi:https://doi.org/10.1093/brain/awu179.
Menon, S., Armstrong, S., Hamzeh, A., Visanji, N.P., Sardi, S.P. and Tandon, A. (2022a). Alpha-Synuclein Targeting Therapeutics for Parkinson’s Disease and Related Synucleinopathies. Frontiers in Neurology, 13(PMC9124903). doi:https://doi.org/10.3389/fneur.2022.852003.
Menon, S., Armstrong, S., Hamzeh, A., Visanji, N.P., Sardi, S.P. and Tandon, A. (2022b). Alpha-Synuclein Targeting Therapeutics for Parkinson’s Disease and Related Synucleinopathies. Frontiers in Neurology, 13(PMC9124903). doi:https://doi.org/10.3389/fneur.2022.852003.
Menon, S., Armstrong, S., Hamzeh, A., Visanji, N.P., Sardi, S.P. and Tandon, A. (2022c). Alpha-Synuclein Targeting Therapeutics for Parkinson’s Disease and Related Synucleinopathies. Frontiers in Neurology, 13. doi:https://doi.org/10.3389/fneur.2022.852003.
Milone, M.C. and O’Doherty, U. (2018). Clinical use of lentiviral vectors. Leukemia, [online] 32(7), pp.1529–1541. doi:https://doi.org/10.1038/s41375-018-0106-0.
Moore, W. (2022). What Is CRISPR? What Conditions Does It Treat? [online] WebMD. Available at: https://www.webmd.com/cancer/guide/crispr-facts-overview.
Morgan, R.A., Gray, D., Lomova, A. and Kohn, D.B. (2017). Hematopoietic Stem Cell Gene Therapy: Progress and Lessons Learned. Cell stem cell, [online] 21(5), pp.574–590. doi:https://doi.org/10.1016/j.stem.2017.10.010.
Mulholland, E.J. (2020). Parkinson’s Disease: Is Gene Therapy the Answer We Have Been Looking For? | ASGCT – American Society of Gene & Cell Therapy. [online] asgct.org. Available at: https://asgct.org/publications/news/april-2020/parkinsons-disease-awareness-month#:~:text=Gene%20therapy%20has%20already%20shown [Accessed 25 Feb. 2023].
Munis, A.M. (2020). Gene Therapy Applications of Non-Human Lentiviral Vectors. Viruses, 12(10), p.1106. doi:https://doi.org/10.3390/v12101106.
Muramatsu, S., Fujimoto, K., Kato, S., Mizukami, H., Asari, S., Ikeguchi, K., Kawakami, T., Urabe, M., Kume, A., Sato, T., Watanabe, E., Ozawa, K. and Nakano, I. (2010). A Phase I Study of Aromatic L-Amino Acid Decarboxylase Gene Therapy for Parkinson’s Disease. Molecular Therapy, [online] 18(9), pp.1731–1735. doi:https://doi.org/10.1038/mt.2010.135.
National Cancer Institute (2011). Adenovirus Vector. [online] www.cancer.gov. Available at: https://www.cancer.gov/publications/dictionaries/cancer-drug/def/adenovirus-vector.
Neves, V., Aires-da-Silva, F., Corte-Real, S. and Castanho, M.A.R.B. (2016). Antibody Approaches To Treat Brain Diseases. Trends in Biotechnology, 34(1), pp.36–48. doi:https://doi.org/10.1016/j.tibtech.2015.10.005.
Norsted, E., Gömüç, B. and Meister, B. (2008). Protein components of the blood-brain barrier (BBB) in the mediobasal hypothalamus. Journal of Chemical Neuroanatomy, [online] 36(2), pp.107–121. doi:https://doi.org/10.1016/j.jchemneu.2008.06.002.
Noyce, A.J., Bestwick, J.P., Silveira-Moriyama, L., Hawkes, C.H., Giovannoni, G., Lees, A.J. and Schrag, A. (2012). Meta-analysis of early nonmotor features and risk factors for Parkinson disease. Annals of Neurology, [online] 72(6), pp.893–901. doi:https://doi.org/10.1002/ana.23687.
Nutt, J.G., Burchiel, K.J., Comella, C.L., Jankovic, J., Lang, A.E., Laws, E.R., Lozano, A.M., Penn, R.D., Simpson, R.K., Stacy, M. and Wooten, G.F. (2003). Randomized, double-blind trial of glial cell line-derived neurotrophic factor (GDNF) in PD. Neurology, 60(1), pp.69–73. doi:https://doi.org/10.1212/wnl.60.1.69.
Oczkowska, A., Kozubski, W., Lianeri, M. and Dorszewska, J. (2013). Mutations in PRKN and SNCA Genes Important for the Progress of Parkinson’s Disease. Current Genomics, [online] 14(8), pp.502–517. Available at: https://www.ingentaconnect.com/content/ben/cg/2013/00000014/00000008/art00003 [Accessed 24 Feb. 2023].
Oertel, W. and Schulz, J.B. (2016). Current and experimental treatments of Parkinson disease: A guide for neuroscientists. Journal of Neurochemistry, 139, pp.325–337. doi:https://doi.org/10.1111/jnc.13750.
Oh, S.E., Park, H.-J., He, L., Skibiel, C., Junn, E. and Mouradian, M.M. (2018). The Parkinson’s disease gene product DJ-1 modulates miR-221 to promote neuronal survival against oxidative stress. Redox Biology, 19, pp.62–73. doi:https://doi.org/10.1016/j.redox.2018.07.021.
OMICS International (2019). Advantages and disadvantages. [online] Omicsonline.org. Available at: https://www.omicsonline.org/articles-images/2161-0932-S6-001-t001.html.
Outeiro, T.F., Koss, D.J., Erskine, D., Walker, L., Kurzawa-Akanbi, M., Burn, D., Donaghy, P., Morris, C., Taylor, J.-P., Thomas, A., Attems, J. and McKeith, I. (2019). Dementia with Lewy bodies: an update and outlook. Molecular Neurodegeneration, [online] 14(1). doi:https://doi.org/10.1186/s13024-019-0306-8.
Oxford Biomedica PLC (2008). Positive results in Phase I/II trial | Oxford Biomedica. [online] www.oxb.com. Available at: https://www.oxb.com/investors/regulatory-news/positive-results-phase-iii-trial [Accessed 20 Feb. 2023].
Palfi, S., Gurruchaga, J.M., Lepetit, H., Howard, K., Ralph, G.S., Mason, S., Gouello, G., Domenech, P., Buttery, P.C., Hantraye, P., Tuckwell, N.J., Barker, R.A. and Mitrophanous, K.A. (2018). Long-Term Follow-Up of a Phase I/II Study of ProSavin, a Lentiviral Vector Gene Therapy for Parkinson’s Disease. Human Gene Therapy Clinical Development, 29(3), pp.148–155. doi:https://doi.org/10.1089/humc.2018.081.
Palfi, S., Gurruchaga, J.M., Ralph, G.S., Lepetit, H., Lavisse, S., Buttery, P.C., Watts, C., Miskin, J., Kelleher, M., Deeley, S., Iwamuro, H., Lefaucheur, J.P., Thiriez, C., Fenelon, G., Lucas, C., Brugières, P., Gabriel, I., Abhay, K., Drouot, X. and Tani, N. (2014). Long-term safety and tolerability of ProSavin, a lentiviral vector-based gene therapy for Parkinson’s disease: a dose escalation, open-label, phase 1/2 trial. The Lancet, 383(9923), pp.1138–1146. doi:https://doi.org/10.1016/s0140-6736(13)61939-x.
Palfi, S., Watts, C., Gurrachaga, J.-M., Buttery, P., Ralph, S., Deeley, S., Lepetit, H., Miskin, J., Iwamuro, H., Lavisse, S., Kas, A., Ramelli, A.L., Tani, N., Dolphin, P., Abhay, K., Gabriel, I., Fenelon, G., Brugiere, P., Naylor, S. and Hantraye, P. (2012). 56. ProSavin® a Gene Therapy Approach for Parkinson’s Disease: Phase I Clinical Trial Update. Molecular Therapy, 20, p.S23. doi:https://doi.org/10.1016/s1525-0016(16)35860-9.
Parkinson’s Foundation (2017). Genetics and Parkinson’s. [online] Parkinson’s Foundation. Available at: https://www.parkinson.org/understanding-parkinsons/causes/genetics.
Parr-Brownlie, L.C., Bosch-Bouju, C., Schoderboeck, L., Sizemore, R.J., Abraham, W.C. and Hughes, S.M. (2015). Lentiviral Vectors as Tools to Understand Central Nervous System Biology in Mammalian Model Organisms. [online] Frontiers in Molecular Neuroscience. Available at: https://www.frontiersin.org/articles/10.3389/fnmol.2015.00014/full#:~:text=Lentiviruses%20have%20been%20extensively%20used,in%20the%20adult%20mammalian%20brain [Accessed 16 Feb. 2023].
Poewe, W., Antonini, A., Zijlmans, J.C., Burkhard, P.R. and Vingerhoets, F. (2010). Levodopa in the treatment of Parkinson’s disease: an old drug still going strong. Clinical Interventions in Aging, [online] 5(229–238), pp.229–238. doi:https://doi.org/10.2147/cia.s6456.
PubChem (2023). Homology Directed Repair. [online] pubchem.ncbi.nlm.nih.gov. Available at: https://pubchem.ncbi.nlm.nih.gov/pathway/Reactome:R-HSA-5693538.
Reeve, A., Simcox, E. and Turnbull, D. (2014). Ageing and Parkinson’s disease: Why Is Advancing Age the Biggest Risk factor? Ageing Research Reviews, [online] 14(100), pp.19–30. doi:https://doi.org/10.1016/j.arr.2014.01.004.
Roach, D. and Lewis, T. (2015). CRISPR gene editing explained. [online] Business Insider. Available at: https://www.businessinsider.com/crispr-gene-editing-explained-2015-12.
Salvatore, M., Ai, Y., Fischer, B., Zhang, A., Grondin, R., Zhang, Z., Gerhardt, G. and Gash, D. (2006). Point source concentration of GDNF may explain failure of phase II clinical trial. Experimental Neurology, 202(2), pp.497–505. doi:https://doi.org/10.1016/j.expneurol.2006.07.015.
Sancho-Bielsa, F.J. (2022). Parkinson’s disease: Present and future of cell therapy. Neurology Perspectives, 2(S58–S68), pp.S58–S68. doi:https://doi.org/10.1016/j.neurop.2021.07.006.
Schlimgen, R., Howard, J., Wooley, D., Thompson, M., Baden, L.R., Yang, O.O., Christiani, D.C., Mostoslavsky, G., Diamond, D.V., Duane, E.G., Byers, K., Winters, T., Gelfand, J.A., Fujimoto, G., Hudson, T.W. and Vyas, J.M. (2016). Risks Associated With Lentiviral Vector Exposures and Prevention Strategies. Journal of Occupational and Environmental Medicine, [online] 58(12), pp.1159–1166. doi:https://doi.org/10.1097/JOM.0000000000000879.
Shen, Y., Muramatsu, S.I., Ikeguchi, K., Fujimoto, K.I., Fan, D.S., Ogawa, M., Mizukami, H., Urabe, M., Kume, A., Nagatsu, I., Urano, F., Suzuki, T., Ichinose, H., Nagatsu, T., Monahan, J., Nakano, I. and Ozawa, K. (2000). Triple transduction with adeno-associated virus vectors expressing tyrosine hydroxylase, aromatic-L-amino-acid decarboxylase, and GTP cyclohydrolase I for gene therapy of Parkinson’s disease. Human Gene Therapy, [online] 11(11), pp.1509–1519. doi:https://doi.org/10.1089/10430340050083243.
Sinclair, A., Islam, S. and Jones, S. (2018). Gene Therapy: An Overview of Approved and Pipeline Technologies. [online] Nih.gov. Available at: https://www.ncbi.nlm.nih.gov/books/NBK538378/ [Accessed 25 Feb. 2023].
Spencer, B.J. and Verma, I.M. (2007). Targeted delivery of proteins across the blood–brain barrier. Proceedings of the National Academy of Sciences, 104(18), pp.7594–7599. doi:https://doi.org/10.1073/pnas.0702170104.
Spillantini, M.G., Crowther, R.A., Jakes, R., Hasegawa, M. and Goedert, M. (1998). -Synuclein in filamentous inclusions of Lewy bodies from Parkinson’s disease and dementia with Lewy bodies. Proceedings of the National Academy of Sciences, 95(11), pp.6469–6473. doi:https://doi.org/10.1073/pnas.95.11.6469.
Stefanis, L. (2011). α-Synuclein in Parkinson’s Disease. Cold Spring Harbor Perspectives in Medicine, [online] 2(2), pp.a009399–a009399. doi:https://doi.org/10.1101/cshperspect.a009399.
Tan, L.C.S. (2013). Epidemiology of Parkinson’s disease. Neurology Asia, [online] 18(3), pp.231–238. Available at: https://www.neurology-asia.org/articles/neuroasia-2013-18(3)-231.pdf.
Tang, Y., Han, T., Everts, M., Zhu, Z.B., Gillespie, G.Y., Curiel, D.T. and Wu, H. (2006). Directing adenovirus across the blood–brain barrier via melanotransferrin (P97) transcytosis pathway in an in vitro model. Gene Therapy, 14(6), pp.523–532. doi:https://doi.org/10.1038/sj.gt.3302888.
Tereshchenko, J., Maddalena, A., Bähr, M. and Kügler, S. (2014). Pharmacologically controlled, discontinuous GDNF gene therapy restores motor function in a rat model of Parkinson’s disease. Neurobiology of Disease, 65, pp.35–42. doi:https://doi.org/10.1016/j.nbd.2014.01.009.
Todorova, A., Jenner, P. and Ray Chaudhuri, K. (2014). Non-motor Parkinson’s: integral to motor Parkinson’s, yet often neglected. Practical Neurology, 14(5), pp.310–322. doi:https://doi.org/10.1136/practneurol-2013-000741.
van Rumund, A., Pavelka, L., Esselink, R.A.J., Geurtz, B.P.M., Wevers, R.A., Mollenhauer, B., Krüger, R., Bloem, B.R. and Verbeek, M.M. (2021). Peripheral decarboxylase inhibitors paradoxically induce aromatic L-amino acid decarboxylase. npj Parkinson’s Disease, 7(1). doi:https://doi.org/10.1038/s41531-021-00172-z.
Wakabayashi, K., Tanji, K., Mori, F. and Takahashi, H. (2007). The Lewy body in Parkinson’s disease: Molecules implicated in the formation and degradation of α-synuclein aggregates. Neuropathology, 27(5), pp.494–506. doi:https://doi.org/10.1111/j.1440-1789.2007.00803.x.
Weintraub, D., Comella, C.L. and Horn, S. (2008). Parkinson’s disease–Part 1: Pathophysiology, symptoms, burden, diagnosis, and assessment. The American Journal of Managed Care, [online] 14(2 Suppl), pp.S40-48. Available at: https://pubmed.ncbi.nlm.nih.gov/18402507/.
Wold, W.S.M. and Toth, K. (2013). Adenovirus Vectors for Gene Therapy, Vaccination and Cancer Gene Therapy. Current Gene Therapy, [online] 13(6), pp.421–433. Available at: https://www.ncbi.nlm.nih.gov/pmc/articles/PMC4507798/.
Wood, M.J.A., Charlton, H.M., Wood, K.J., Kajiwara, K. and Byrnes, A.P. (1996). Immune Responses to Adenovirus Vectors in the Nervous System. Trends in Neurosciences, [online] 19(11), pp.497–501. doi:https://doi.org/10.1016/S0166-2236(96)10060-6.
Xie, F.Y., Woodle, M.C. and Lu, P.Y. (2006). Harnessing in vivo siRNA delivery for drug discovery and therapeutic development. Drug Discovery Today, 11(1-2), pp.67–73. doi:https://doi.org/10.1016/s1359-6446(05)03668-8.
Zhang, Y. and Pardridge, W.M. (2008). Near Complete Rescue of Experimental Parkinson’s Disease with Intravenous, Non-viral GDNF Gene Therapy. Pharmaceutical Research, 26(5), pp.1059–1063. doi:https://doi.org/10.1007/s11095-008-9815-9.+