Supervised by: Amanda Liu, BEng, MSc. Amanda spent her undergraduate years studying Biochemical Engineering at UCL (University College London), where she was awarded First Class Honours. She then completed her Master’s degree in Clinical and Therapeutic Neuroscience at the University of Oxford. She is currently studying Medicine (Graduate Entry) at the University of Cambridge.
Abstract
40% of all spinal cord injury cases occur between the c4,c5 and c6 vertebrae (1). The possible results of an injury in that zone include paralysis in the arms, hands, torso and legs, other than affected gray-to-white matter ratio (2). One day, being able to reverse these damages should be possible. In 2009 an article released by Jason Sharp, Jennifer Frame, Monica Siegenthaler, Gabriel Nistor, and Hans S. Keirstead talked about how Human Embryonic Stem Cell-Derived Oligodendrocyte Progenitor Cell Transplants improve recovery after cervical spinal cord injury. What was achieved by this treatment is remyelination of the damaged axons, which were demyelinated other than robust white and gray matter starting in the injury’s epicenter and, most importantly, preservation of motor neurons that correlated with movement recovery. Rats are injected with hESC-derived OPCs in the hope that these will mature into oligodendrocytes and achieve remyelination. This innovative treatment involving human stem cells is yet to be tested on humans, but the testing conducted on rats has produced positive results.
Introduction
Spinal cord injury involves the injury of the tight bundle of cells and nerves located in the spinal cord. This damage is caused by direct insult to the spinal cord or the tissue and bones around the spinal cord. Spinal cord injury currently affects 296,000 people in the United States alone. The most common causes of spinal cord injuries come from motor vehicle accidents, violent acts, sports injuries and diseases. Symptoms of spinal cord injury vary depending on the severity of injury and location on the spinal cord. Some symptoms include loss of sensory function or motor control of the body and chronic pain. The most severe injury damages systems managing bowel or bladder control, breathing and blood circulation. As a result, finding a treatment that allows patients to recover from spinal cord injury is essential.
As the adult nervous system has low regenerative capabilities, much research focuses on improving the regeneration of and reducing the degeneration of the CNS. Stem cells are largely targeted to address this challenge as they allow for the replacement or recovery of damaged cells in the spinal cord. Spinal cord injury results in the death of oligodendrocytes, cells that are able to produce myelin and assist the long-term integrity of myelin, and damages axons by removing the myelin sheath at the site of trauma. Demyelination is associated with a long list of CNS pathologies. This paper investigates and discusses a study done on the transplantation of oligodendrocytes progenitor cells (OPCs) derived from human embryonic stem cells (hESCs) at the site of injury as it is a primary target to promote recovery in the spinal cord by remyelination of axons.
Methods
Spinal Cord Injury
Cervical spinal cord contusion injuries were performed on female Sprague Dawley adult rats (200–220 g) as described [14]. A dorsal laminectomy was performed on the fifth cervical vertebra (C5) to expose the spinal cord. The rat was suspended by clamps on the vertebrae cranial and caudal to the laminectomy. A contusion injury was induced with a force of 200 kDyn (n = 46). After injury, both deep and superficial muscle layers were stitched, and the skin was closed with stainless-steel wound clips. Immediately after surgery, the rats were given subcutaneous saline and Baytril (2.5 mg/kg/d) and retained on an isothermal pad until alert and capable of movement. Animals received manual bladder expression twice daily and were inspected for weight loss, dehydration, and distress, with appropriate veterinary care when needed.
Derivation Of Oligodendrocyte Progenitor Cells
The H7 hESC line was obtained from the Geron Corporation. Cells were expanded in hESC growth media [23] and differentiated according to published protocols [18, 24, 25]. Briefly, dissociated colonies were placed into low attachment flasks in 50% hESC growth media and 50% glial restriction media for 2 days. On day 1, the media was supplemented with 4 ng/ml of basic fibroblast growth factor and 20 ng/ml of epidermal growth factor. On day 2, it was supplemented with epidermal growth factor and 10 μM all-trans-retinoic acid. This media was replaced with 100% glial restriction media supplemented with epidermal growth factor and all-trans-retinoic acid for an additional 7 days.
Cells were then exposed for 18 days to glial restriction media/epidermal growth factor without all-trans-retinoic acid. On day 28, yellow spheres were plated in flasks coated with 1:30 Matrigel for 7 days. Cultures were then trypsinized, remnant spheres were excluded, and cells were replated on Matrigel and cultured for 7 days in glial restriction media/epidermal growth factor. The total time for the differentiation protocol was 42 days.
BT
Forelimb movement scores were determined from videotapes of the rats crossing a clear Plexiglas walkway marked with 1 cm grid lines on the floor. Before testing, each rat was acclimated to the environment and trained to cross for intermittent food rewards. During test trials, animals were videotaped from underneath and from the side to properly examine their approximate forelimb step range, forelimb stride length, and passed-perpendicular step frequency of the walkway using a Canon video camera. Videos were analyzed frame by frame using media player software.
To analyze forelimb stride length, the rats were taped from below the walkway each week from weeks 1–3 and then every other week from weeks 5–9. The videos were scored for forelimb stride length as outlined for hindlimb analysis by Gonzalez et al. [26]. Forelimb stride length was roughly defined as the distance between the start and end of a step with either one of the rat’s forepaws. Measurements were taken on each side for five steps and averaged for each animal.
Transplant
Both groups received cyclosporine A (in doses of 20 mg/kg/d) 1 day before transplantation and then every day for the duration of the study to reduce the chances of immune rejection. Cell transplantation occurred 7 days after injury, the animals were anesthetized, and the laminectomy site was re-exposed.
Following immobilization of the spinal process cranial to the contusion site, a 10 μl Hamilton syringe was lowered into the spinal cord using a stereotactic manipulator arm. Cell suspensions were injected along the midline of the spinal cord at a depth of 1.2 mm into one site cranial and one site caudal to the lesion epicenter, in a total volume of 7.5 μl, containing approximately 1,500,000 cells, at a rate of 1 μl/minutes. Control animals received an equal volume of vehicle at the same injection rate.
Total RNA was isolated from cultured OPCs using Trizol reagent (dissolves cell material) followed by DNAse I digestion (kills off living components of a cell) and finally RNeasy (RNA extractor). Reverse transcription of mRNAs was performed using M-MLV Reverse Transcriptase with random hexamers and poly-dT used as primers. cDNAs generated by reverse transcription were used for subsequent 30-cycle PCRs (a method used to generate copies of DNA) using a Mastercycler thermal cycler, Platinum Taq DNA polymerase, and specific primers for TGFβ1 (protein that performs functions such as cell growth and proliferation). PCR products were analyzed by gel electrophoresis.
Histology
Animals were killed 8 weeks after cell transplantation under pentobarbital anesthesia by aortic perfusion with isotonic, heparinized saline followed with 4% paraformaldehyde in 0.1 M phosphate buffer, pH 7.4. The spinal cord was split into eight 1 mm blocks that extended 4 mm cranial to and 4 mm caudal to the injury epicenter. Alternate blocks were processed to produce resin or cryostat sections. Resin sections were used to determine the number of myelinated axons, the gross pathology of the transplant environment, and morphometric measurements and cryostat sections were used for immunodetection.
For resin processing, blocks were postfixed for 24 h in 4% glutaraldehyde and embedded in resin according to standard protocols. Transverse semithin 1 μm sections were cut from the cranial face, stained with alkaline toluidine blue, coverslipped, and examined by light microscopy on an Olympus AX-80 microscope and a 2-megapixel MagnaFire digital camera using Olympus MicroSuite B3SV software. For electron microscopy, blocks were trimmed and sections were cut at 100 nm, mounted on copper grids, uranyl acetate, and lead citrate stained, and viewed under a Hitachi EM 600 electron microscope at 75 kV.
Morphometry
Morphometric analysis was performed as described [14]. To quantify naturally myelinated, demyelinated, and oligodendrocyte remyelinated axons, regions of pathology were located on 1 μm resin sections at 40x magnification and delineated using Olympus MicroSuite B3SV software to calculate perimeter and area as reported [14]. Normally myelinated, demyelinated, and oligodendrocyte remyelinated axons were measured using a systematic line-sampling technique also used by Blight AR [27]. The average number of normally myelinated, demyelinated, and oligodendrocyte- or SC-remyelinated axons within five 25 μm2 × 25 μm2 areas along the radial line produced an estimate of the total number of axons within a region of pathology and was calculated as the number of axons per square millimeter. At least 10% of the area of pathology is used to determine the total axons for each area of pathology. The number of oligodendrocyte-remyelinated and demyelinated axons was used to determine the oligodendrocyte-remyelination efficiency.
Myelin Pathology Counts
Briefly, 1 μm transverse semithin spinal cord sections were imaged at 40x using an Olympus AX-80 microscope and 2-megapixel MagnaFire digital camera, and measurements were traced on the image using the Olympus MicroSuite B3SV software to determine area and perimeter values. Spared white matter area was marked by less than 50% pathology that included aberrant hallmarks such as swelling, damaged axons, hypercellularity, and gross demyelination. Multiple areas of pathology within a section were individually traced and then summed. Spared gray matter area was defined as gray matter area(s) that were clearly identifiable from white matter. The measured perimeters per section were used to derive the value of the maximal area for each parameter, as detailed by Schrimsher and Reier [15].
The measured perimeters per section were used to derive the value of the maximal area for each parameter, and the maximal area was used for comparisons between animals to account for potential tissue processing artifacts.
Statistical Methods
Forelimb stride length scores were analyzed using two-way, repeated measures ANOVA with Tukey’s multiple comparison test at each time point. The treatment group (transplant versus control) was set as the between-groups factor, and the weak interval was set as the within-group, repeated-measures factor. The Statistical Package for the Social Sciences 11.5 t-test was used to determine differences between proximal forelimb range scores for transplanted and control groups. The SPSS 11.5 t-test was used to determine differences between quantitative histological and qPCR values.
Results
Differentiation
At the end of the 42-day differentiation protocol, hESCs (Embryonic Stem Cells) started to get the Bipolar pattern and shape of immature OPCs. They also had a typical antigenic profile, meaning they were immunopositive to markers of genes present in certain types of Oligodendrocytes:
- >90% to NG2
- >80% to Olig1
- >70% to PDGFRα
- <5% to A2B5
- <5% of Tuj1 neuronal cells
- <1% of GFAP-positive astrocytes
No more-mature oligodendrocyte markers such as O4 or RIP were positive.
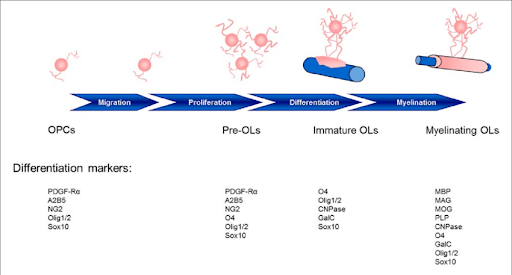
Stages of maturation of Oligodendrocytes and the gene markers to identify them. https://www.researchgate.net/figure/Stages-of-oligodendrocyte-maturation-toward-the-oligodendroglial-lineage_fig2_294285000
HESCs’ genes Oct-4 and SSEA4 were not found positive in any cells, meaning that no hESC were left behind.
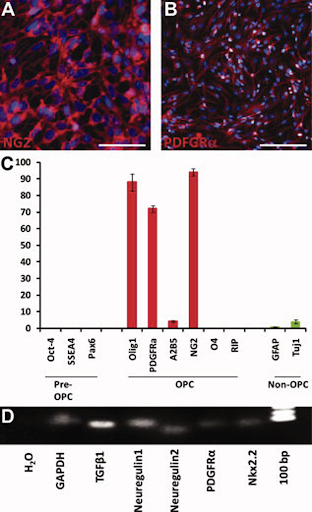
OPCs coming from injected hESCs expression profile. A) 90% of cells with the NG2 protein in red. B) Total of 72±2% of cells showed the cell surface tyrosine kinase receptor for platelet-derived growth factor, PDGFRα, shown in red. C) The black bars show the absence of the embryonic stem cell markers Oct-4 and SSEA4 and neural development transcription factor Pax6. C) Fewer than 5% of cells show the presence of the marker A2B5, found in less mature OPCs or the more mature OPC marker, O4 and RIP (red bars). C) Less than 5% of cells are immunopositive to markers GFAP and Tuj1, which are not typical of OPCs. D) Using reverse transcription-polymerase chain reaction (turning RNA in DNA) shows the detected mRNA.
Also detected were proteins secreted with different functions, including mediating differentiation, such as TGFβ1, Neuregulin 1, and Neuregulin 2.
Bilateral Cervical Contusion Pathology
The animals suffered from a contusion that showed functional preservation of cranial phrenic motor neuron pools. One week after the injury animals regained bladder function and were able to access water and food on their own.
Animals without the transplanted spinal cord were checked on 9 weeks after. The results of this show a bilateral cavitation with center at the impact site that extended approximately 2 mm along the cranial-caudal axis. Examinations showed the maximal area of cavitation being at the injury epicenter. Morphometric analysis at the epicenter showed that the maximal area of the cavity comprised approximately 18% of the total area of the spinal cord. The injured spinal cord showed marked contusion pathology that included gray matter loss, axonopathy, scattered demyelination, oligodendrocyte-remyelination, partitioned Schwann cell (SC)-remyelination, inflammatory infiltrates, and perivascular cuffing.
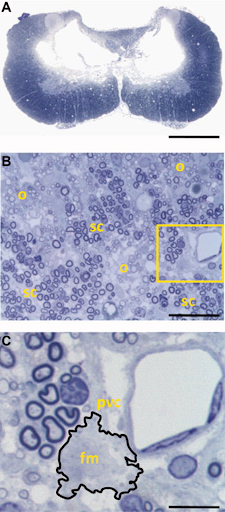
Anatomical features of midline, bilateral cervical contusion. A) Toluidine blue-stained section shows loss of bundles of neuron cell bodies (gray matter) and bundles of axons (white matter) at the injury epicenter. This involved interneurons of laminae III-IV and some loss of interneurons and motor neurons laminae II, VII and VIII. B) White matter pathology, including scattered demyelination, oligodendrocyte remyelination (o), and partitioned Schwann cell remyelination (sc). Inspection of vasculature within areas of white matter pathology (yellow box, (B)) identified foamy macrophages (outlined) associated with capillaries, suggestive of ongoing pathology. C) pvc shows perivascular cuffing (inflammation around a blood vessel); foamy macrophages. Scale bar: a, 1 mm; b, 40 μm; c, 4 μm.
Transplanted OPCs Survive, Localize to Injury, and Differentiate
Transplanted hESC-derived OPCs managed to localize the injury site and get there alive during the study period of 9 weeks. At the injury epicenter, anti-human cells were identified throughout the whole plain, but were largely concentrated around the area of cavitation. There were also some other rodent cells identified around the site, shown by the methyl green-only staining in figure 3B. Quantitation of human nuclei revealed the highest number of cells at the injury epicenter and very few cells cranial and caudal to the epicenter as shown in figure 3C.
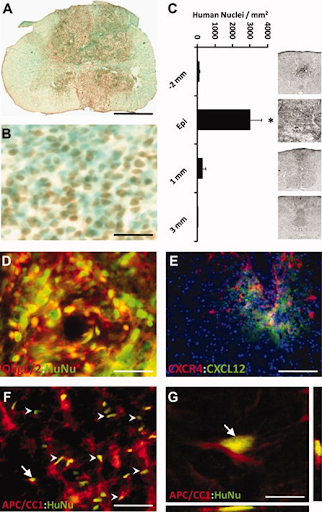
Transplantation of human embryonic stem cell (hESC)-derived oligodendrocyte progenitor cells (OPCs) into acute cervical spinal cord injury resulted in cell survival, limited migration from the site of implantation, and differentiation to mature oligodendrocytes. A) hESC-derived OPCs were detected using anti-human-positive nuclei staining (brown), shows the difference with control rats with no human cells detected.B) Close image of the transplant area reveals non-human cells (blue-green) among the anti-human nuclei-positive, transplanted cells (brown). Within the transplant area, human nuclei positive cells were homogeneously distributed and were not located in discrete clusters or rosette-like formations in any transplanted animals as might suggest neuroblastoma/glioma. C) Distribution of total numbers of anti-human nuclei-positive cells within spinal cord transverse sections along the cranial-caudal axis. Cells were very concentrated towards the injury epicenter. Inset panels illustrate limited distribution of transplanted OPCs (black punctuation) in the dorsal column. D) Clusters of anti-human nuclei-positive cells (green) double labeled with Olig1 (red) within a region of high concentration of human nuclei-positive cells. E) 2–3 mm from the injury epicenter, human-specific CXCR4 (red) cells localized to sites of rat/mouse-specific CXCL12 (green), suggesting that the transplanted cells responded to host cell-migration cues. F) Anti-human nuclei-positive cells (HuNU, green) double-labeled (arrows) with the mature oligodendrocyte marker APC/CC1 (red) detected within a region of white matter. Endogenous APC/CC1-positive oligodendrocytes were present within the white matter of transplants and controls. G) 3-D reconstruction of confocal scanned thin-planed images (arrows is F and G) confirmed double-labeling of anti-human nuclei (HuNu, green) and APC/CC1 (red). No human cells were found that co-labelled with the astrocytes marker, GFAP, the neuronal marker, NeuN, or the pluripotent marker, Oct-4 (data not shown). Scale bar: a, 1.25 mm; b, d, 45 μm; e, 200 μm; f, 90 μm; g, 8 μm.
Human-nuclei positive cells in figure 3F show that transplanted OPCs became oligodendrocytes in regions appropriate for myogenic differentiation.
OPC Transplantation Improves Forelimb Motor Function After Injury
Both control and transplanted groups of rats’ forelimb stride lengths were examined. By 4 weeks post-transplant, the transplanted rats showed a significantly longer stride in comparison to the control rats. The stride length of the control group peaked at 1-week post-transplant, contrasting with the transplanted group’s stride, which peaked 6 weeks post-transplant. From this, it can be concluded that the transplanted group has shown an extensive recovery before reaching the highest level of functionality.
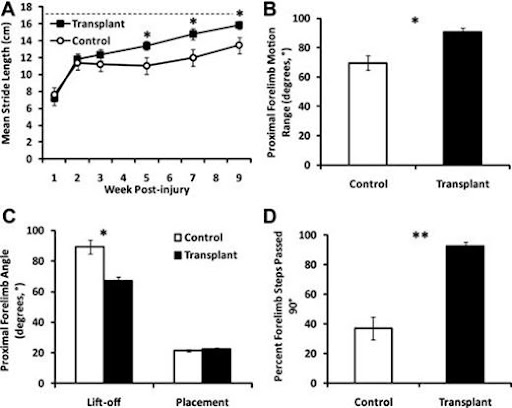
Improvements made to forelimb motion after the transplant of hESC)-derived oligodendrocyte progenitor cell (OPC). A) Shows how transplanted animals clearly gained a more significant improvement in forelimb stride length in comparison to control animals (*, p < .01). Transplanted animals nearly managed to get to approximately the same mean stride length of un-injured species trained for the same test (17.5cm, dashed line) B) Range of proximal forelimb motion in degrees was significantly increased in the transplant group compared to the non transplanted group (*, p < .01). C) The increased range of proximal forelimb motion was resolved to the lift-off (rearmost) position of the step as opposed to the placement (foremost) by measurement of the inside angle between the forelimb and the floor at lift-off and placement (*, p < .01). D)The occurrence of steps that pass the perpendicular plane is significantly increased from frequent (>50%) in the transplant group versus occasional (≤50%) in the control group (**, p < .001).
OPC Transplantation Alters Lesion Pathogenesis
The injury epicenter within non-transplanted spinal cords exhibited widespread loss of white matter and gray matter (Fig. 5A). Cavity borders lacked cells and axons, consistent with glial scar formation (Fig. 5C). White matter pathology was most evident in the dorsal column and medial ventral white matter (Fig. 5A). Non-transplanted spinal cords contained extensive oligodendrocyte remyelination and partitioned SC-remyelination with scattered demyelination (Fig. 5E). In addition, perivascular cuffing and inflammatory infiltrates were present in the control animals, suggestive of dynamic, ongoing pathology within these focal lesions.
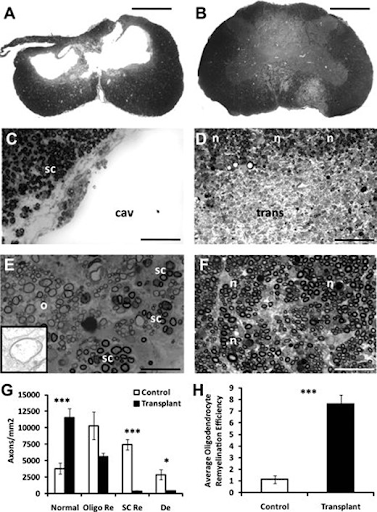
A) Histopathogenesis in transplanted and non-transplanted animals. B) Transplanted animals lack cavitation, and myelin pathology is less extensive compared to non transplanted animals. C)The dorsal column along the cavitation contains mostly Schwann cell (SC)-myelinated axons (sc) with an edge that is consistent with scar formation. D) (n)=normally myelinated axons, (trans)= transplanted cells. No delineation between (trans) and host cells. The dorsal column of transplanted animals contains mostly (n). E) Non-transplanted animals. (o)=oligodendrocyte-remyelination, (sc)=partitioned SC-remyelination. Also shown are a few small-diameter axons. Inset illustrates the thin myelin sheath characteristic of oligodendrocyte-remyelination. F) Shows transplanted animal cord. (n)= spared myelination. More small-diameter axons compared to controls are seen. G) Data shows normally myelinated axons, oligodendrocyte-remyelinated axons, SC-remyelinated axons and unmyelinated axons. These data indicate that normally myelinated and oligodendrocyte-remyelinated axons constitute about 95% of the total axon population within the area of dorsal column pathology in transplanted animals. H) (***, p < .001) shows how transplanted animals are about 6 fold more efficient than control animals when it comes to oligodendrocyte remyelination. Error bars represent ±SEM. Scale bar: a,b, 1.25 mm; c,d, 60 μm; e, 30 μm; f, 40 μm.
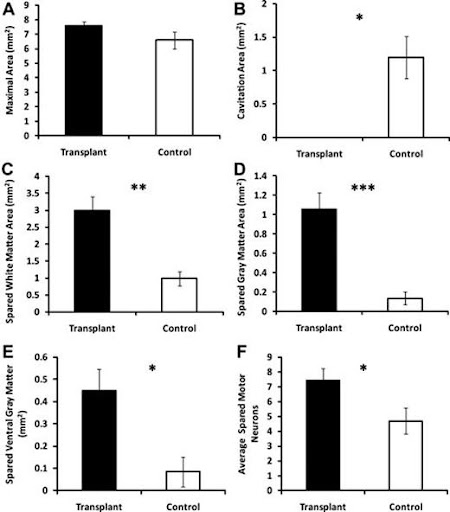
Injury epicenter morphometry and motor neuron counts in transplanted and non transplanted animals. A) No significant difference in maximal area. B) (*, p < .05) cavitation area is significantly decreased in transplanted animals. C) Total spread white matter increased in the transplanted group in comparison to control animals. D) Total spread gray matter increased in the transplanted group in comparison to the control group. E) Also spread ventral gray matter increased in the transplanted group relative to the control group. F) Same thing for spreading motor neurons; increase in transplanted animals in comparison to control animals.
A test was done to test the correlation between functional and histological outcomes, and the results show a significant correlation was found for proximal forelimb range of motion and mean motor neuron sparing (r = .7153, p < .01) and ventral gray matter sparing (r = .6612, p < .05). Together, these data suggest that hESC-derived OPC transplantation can attenuate tissue loss and thereby preserve spinal cord mediated function.
Transplantation of OPCs Affects Gene Expression in Acute Phase of Spinal Cord Injury
21 days post-injury, fold changes were detected as significantly different for select genes (GOIs) for neurotrophic factors, apoptosis, inflammation, and a neuronal cell marker.
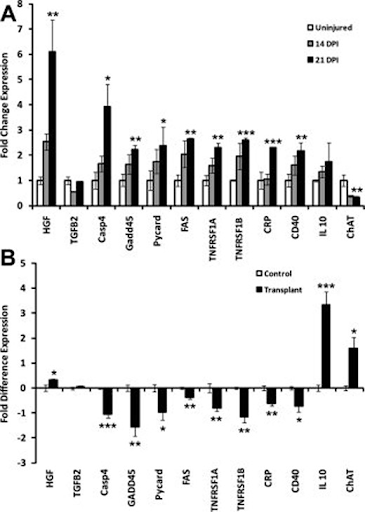
Differential expression of select genes of interest (GOIs) at injury epicenter for neurotrophic factors, apoptosis, inflammation, and neurons in transplanted compared to non transplanted animals. A) Time course of differential expression of select GOIs detected significantly (*, p < .05, **, p < .01, ***, p < .001). Fold change expressions for select GOIs of transplanted group after 14 days post-injury and 21 days post injury. B) 21 days post injury (14 days post transplant) differential gene expression shows significantly (*, p < .05, ***, p < .001) increased fold difference expression of genes for HGF, IL10, and ChAT and significantly (*, p < .05, **, p < .01, ***, p < .001) decreased expression of genes for Casp4, GADD45, Pycard, Fas, TNFR1a, TNFR1b, CRP, and CD40 in transplanted versus non transplanted animals.epicenter morphometry and motor neuron counts in transplanted and non transplanted animals.
21 days after the injury the expression of relevant, specific genes, changed to have a tendency towards an uninjured pattern. Specifically, fold difference expression of genes for HGF (p < .05), IL10 (p < .001), and ChAT (p < .05) were significantly increased, whereas genes for Casp4 (p < .001), GADD45 (p < .01), Pycard (p < .05), Fas (p < .01), TNFR1a (p < .01), TNFR1b (p < .01), CRP (p < .01), and CD40 (p < .05) were significantly decreased.
Discussion
Ultimately, demyelination of axons is a major part of SCI as it leads to symptoms such as loss of function, trauma, stroke, and multiple sclerosis. The basic idea from this is remyelination research, to be able to use human embryonic stem cells (hESCs) to cause regrowth of axons and oligodendrocytes so that loss of nervous tissue function through SCI would be minimized. A drawback of this method is that it would need to be done in the early-post injury period.
There are other drawbacks and positives to Oligodendrocyte Progenitor Cell transplant treatment. For example, in order to make cells progenitor cells, pluripotent cells from high-purity lineages are used. This would, in turn, cause differentiation into teratomas, tumors that develop from pluripotent cells that divide abnormally, like cancer cells, but have multiple body tissue types. How this issue could be combatted is that Progenitor Embryonic stem cells have to be directed through differentiation to specialize into multipotent neural precursors, which can be used to remyelinate damaged oligodendrocytes after SCI.
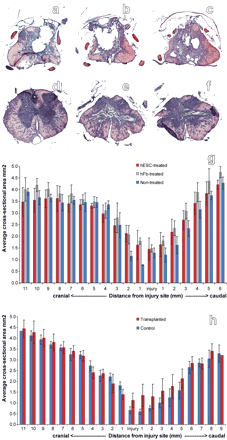
Figure 8. Morphometric analysis indicated that transplantation of hESC-derived OPCs 1 week or 10 months after SCI decreased cavitation but did not alter the extent of tissue sparing or tissue loss. Animals receiving vehicle-only consistently demonstrated cavitation at 1 mm cranial to the injury epicenter (a), at the epicenter (b), and 1 mm caudal to the injury epicenter (c). Animals receiving either OPC transplants consistently demonstrated little or no cavitation at 1 mm cranial to the injury epicenter (d), at the epicenter (e), and 1 mm caudal to the injury epicenter (f). Morphometric analyses at 1 mm intervals through the spinal cord indicated that transplantation of OPCs 7 d (g) or 10 months (h) after SCI did not cause overt tissue sparing or tissue loss. Error bars illustrate SD. Magnification: a-f, 10×.
The above results prove that hESCs that differentiated into OPCs (Oligodendrocyte Progenitor Cells) survived, which provides evidence that in the near future, OPC treatment is viable as results, for now, show that OPCs can function as a replacement for multipotent neural processors without signs of antigenic markers. Not only were the OPCs free of antigenic signs immediately after the test, the differentiated OPCs were observed for a 10-12 month period and the result of remyelinated and resheathed axons was astonishing. SInce the experiment was done in 2 groups the group with the direct OPC transplant increased remyelination levels by almost 136% in comparison to the control group with the endogenous remyelinated axons. In the case of the 2nd group with acute OPC transplantation the result was a 72% increase in the remyelination of axons.
Though OPC treatment that occured in recent SCIs resulted in fascinating amounts of remyelination, if the procedure was done after 10 months of the SCI, remyelination was almost at par with the levels of those in the control group with almost little to none remyelination. The reason for this could be that after a period of time in succession of SCI, Neurons still present in the spine tend to go through progressive demyelination, therefore even with the introduction of OPCs the demyelinated axons in the spinal region might not have remyelinated.
Additionally, deficient remyelination might be a result of astrogliosis and chronic demyelination. Like in the lesioned adult rat anterior medullary velum (Butt and Berry, 2000), the demyelinated guinea pig optic nerve (Sergott et al., 1985), the hypomyelinating taiep mutant rat (Krsulovic et al., 1999), and dysmyelinating mutant mice (Jacque et al., 1986) remyelination occurs in the face of reactive astrocytes. Therefore one of the reasons for remyelination not being proficient after 10 months is that astrogliosis might cause the lack of functionality or astrocytes themselves.
With these studies and finding a plausible conclusion would be that only groups with OPC transplants were able to result in improvement of remyelination, though those transplanted after 10 months showed no improvement, in cases that OPC treatment was administered immediately, remyelination occured and led to developed axons and neurons.
Lastly, a discussion about any discrepancies in the experimental results would be that a rat spine is anatomically different from a human spine, for example, in posture and mass, affecting the bone structure and its components. A rat spine is more slender and curved in comparison to a human spine. Finally, one major difference is that a rat has a 6th lumbar vertebrae while a human has only 5 this would affect the neurological system as it would increase distance and increase the number of neurons needed in order to function normally.
Comparison of results:
Compared to a similar study done by the University of California in 2005, both studies had shown hESC cells that displayed a bipolar morphology at day 42, which is characteristic of immature OPCs. Furthermore, in this test, the cell population for acute transplantation expressed Olig1 (84+-6%) and NG2 (97+-3%), and cell population for chronic transplantation expressed Olig1 (88+-5%) and NG2 (99+-1%) which is similar to the cell population done in the above test. Also, both results showed no SSEA4-positive cells, which shows that all the hESCs had differentiated into astrocytes, neurons or oligodendrocytes.
Besides that, Bisbenzimide-positive, BrdU-positive, and anti-human nuclei positive cells were detected in animals with transplants in both tests which shows that transplanted OPCs are able to live and redistribute in a small distance around site of implantation. Both studies had all control animals not display any of these traits which shows that these results are not caused by the cells within the animal but rather by the transplanted OPCs. Despite both studies using different methods of testing locomotion function, both studies reported that OPCs transplant was able to improve motor function of the animals. In this study, animals received higher scores on locomotor functions. Motor functions also improved in this study as rats transplanted with OPCs had significantly greater rear paw strength and significantly less paw strength width. Overall, this shows that oligodendrocytes differentiated from transplanted OPCs are able to improve motor function in humans by remyelinating damaged axons at location of injury.
Conclusion
Oligodendrocyte progenitor cell transplants truly possess the potential to overcome great medical issues and help improve the quality of life of many people. It may allow those with severe spinal cord ailments to live relatively normal lives and take part in the most menial yet fundamental tasks like spending time with family outside of their medical establishments. Treatments like these take saving and improving lives to the next level while they begin to pave the way for other treatments that will eventually bring humanity into the next generation of healthcare. Medical research is an extraordinarily complicated field that is only made possible by the society of scientists willing to cooperate with one another to utilize their research and technologies in order to benefit the ever-expanding system of people studying, practicing, collaborating, mentoring, helping, and caring for each other in medicine. Now, stem cells are being researched and developed into procedures like OPC transplants that can become the cornerstone of modern medicine. We are faced with the unfortunate reality that sometimes people can injure themselves and only recover to a certain degree, but with enough research, funding, and effort, limits of cell regeneration can be pushed and repair even further. With OPC transplants, there is now a way to replenish nerve cells, cells that cannot multiply on their own and might even be able to fully cure illnesses like paralysis. It is treatments like OPC that will one day change the world and countless people’s lives.
Citation
1) Hagg T, Oudega M. Degenerative and spontaneous regenerative processes after spinal cord injury. J. Neurotrauma 2006;23:264–280. Google Scholar | PubMed | WorldCat
2) Crowe MJ, Bresnahan JC, Shuman SL et al. Apoptosis and delayed degeneration after spinal cord injury in rats and monkeys. Nature Medicine 1997;3:73–76. Google Scholar | CrossrefPubMed | WorldCat
3) Kakulas BA. A review of the neuropathology of human spinal cord injury with emphasis on special features. J Spinal Cord Med 1999;22:119–124. Google Scholar | CrossrefPubMed | WorldCat
4) Ankeny DP, Popovich PG. Mechanisms and implications of adaptive immune responses after traumatic spinal cord injury. Neuroscience 2009;158:1112–1121. Google Scholar | CrossrefPubMed | WorldCat
5) Horky LL, Galimi F, Gage FH et al. Fate of endogenous stem/progenitor cells following spinal cord injury. J Comp Neurol 2006;498:525–538. Google Scholar | CrossrefPubMed | WorldCat
6) Mothe AJ, Tator CH. Proliferation, migration, and differentiation of endogenous ependymal region stem/progenitor cells following minimal spinal cord injury in the adult rat. Neuroscience 2005;131:177–187. Google Scholar | CrossrefPubMed | WorldCat
7) Yamamoto S, Yamamoto N, Kitamura T et al. Proliferation of parenchymal neural progenitors in response to injury in the adult rat spinal cord. Exp Neurol 2001;172:115–127. Google Scholar | CrossrefPubMed | WorldCat
8) Frisén J, Johansson CB, Torok C et al. Rapid, widespread, and long lasting induction of nestin contributes to the generation of glial scar tissue after CNS injury. J Cell Biol 1995;131:453–464. Google Scholar | CrossrefPubMed | WorldCat
9) Faulkner JR, Herrmann JE, Woo MJ et al. Reactive astrocytes protect tissue and preserve function after spinal cord injury. J Neurosci 2004;24:2143–2155. Google Scholar | CrossrefPubMed | WorldCat
10) Silver J, Miller JH. Regeneration beyond the glial scar. Nature Rev 2004;5:146–156. Google Scholar | Crossref | WorldCat
11) NSCISC. Facts and Figures at a Glance. Available at: http://www.spinalcord.uab.edu/show.asp?durki=21446.
12) Anderson KD. Targeting recovery: Priorities of the spinal cord-injured population. J Neurotrauma 2004;21:1371–1383. Google Scholar | CrossrefPubMed | WorldCat
13) Pearse DD, Lo TP, Jr., Cho KS et al. Histopathological and behavioral characterization of a novel cervical spinal cord displacement contusion injury in the rat. J Neurotrauma 2005;22:680–702. Google Scholar | CrossrefPubMed | WorldCat
14) Siegenthaler MM, Tu MK, Keirstead HS. The extent of myelin pathology differs following contusion and transection spinal cord injury. J Neurotrauma 2007;24:1631–1646. Google Scholar | CrossrefPubMed | WorldCat
15) Schrimsher GW, Reier PJ. Forelimb motor performance following cervical spinal cord contusion injury in the rat. Exp Neurol 1992;117:287–298. Google Scholar | CrossrefPubMed | WorldCat
16) Gensel JC, Tovar CA, Hamers FP et al. Behavioral and histological characterization of unilateral cervical spinal cord contusion injury in rats. J Neurotrauma 2006;23:36–54. Google Scholar | CrossrefPubMed | WorldCat
17) McKenna JE, Prusky GT, Whishaw IQ. Cervical motoneuron topography reflects the proximodistal organization of muscles and movements of the rat forelimb: a retrograde carbocyanine dye analysis. J Comp Neurol 2000;419:286–296. Google Scholar | CrossrefPubMed | WorldCat
18) Nistor GI, Totoiu MO, Haque N et al. Human embryonic stem cells differentiate into oligodendrocytes in high purity and myelinate after spinal cord transplantation. Glia 2005;49:385–396. Google Scholar | CrossrefPubMed |WorldCat
19) Keirstead HS, Nistor G, Bernal G et al. Human embryonic stem cell-derived oligodendrocyte progenitor cell transplants remyelinate and restore locomotion after spinal cord injury. J Neurosci 2005;25:4694–4705. Google Scholar | CrossrefPubMed | WorldCat
20) Cloutier F, Siegenthaler MM, Nistor G et al. Transplantation of human embryonic stem cell-derived oligodendrocyte progenitors into rat spinal cord injuries does not cause harm. Regen Med 2006;1:469–479. Google Scholar | CrossrefPubMed | WorldCat
21) Faulkner J, Keirstead HS. Human embryonic stem cell-derived oligodendrocyte progenitors for the treatment of spinal cord injury. Transpl Immunol 2005;15:131–142. Google Scholar | CrossrefPubMed | WorldCat
22) Zhang YW, Denham J, Thies RS. Oligodendrocyte progenitor cells derived from human embryonic stem cells express neurotrophic factors. Stem Cells 2006;15:943–952. Google Scholar | Crossref | WorldCat
23) Carpenter MK, Inokuma MS, Denham J et al. Enrichment of neurons and neural precursors from human embryonic stem cells. Exp Neurol 2001;172:383–397. Google Scholar | CrossrefPubMed | WorldCat
24) Hatch MN, Nistor GI, Keirstead HS. Oligodendrocyte Differentiation from Human Embryonic Stem Cells. In:Loring J, Wessel Schmidt RL, Schwartz PH, eds.Human Stem Cell Manual: A Laboratory Guide, 1st ed. New York:Elsevier;2007:210–226. Google Scholar | Crossref | Google Preview | WorldCat | COPAC
25) Hatch MN, Nistor G, Keirstead HS. Derivation of high-purity oligodendroglial progenitors. Methods Mol Biol 2009;549:59–75. Google Scholar | CrossrefPubMed | WorldCat
26) Gonzalez R, Glaser J, Liu MT et al. Reducing inflammation decreases secondary degeneration and functional deficit after spinal cord injury. Exp Neurol 2003;184:456–463. Google Scholar | CrossrefPubMed | WorldCat
27) Blight AR. Cellular morphology of chronic spinal cord injury in the cat: Analysis of myelinated axons by line-sampling. Neuroscience 1983;10:521–543. Google Scholar | CrossrefPubMed | WorldCat
28) Schmittgen TD, Livak KJ. Analyzing real-time PCR data by the comparative C(T) method. Nat Protoc 2008;3:1101–1108. Google Scholar | CrossrefPubMed | WorldCat
29) Schaal SM, Kitay BM, Cho KS et al. Schwann cell transplantation improves reticulospinal axon growth and forelimb strength after severe cervical spinal cord contusion. Cell Transplant 2007;16:207–228. Google Scholar | CrossrefPubMed | WorldCat
30) Eftekharpour E, Karimi-Abdolrezaee S, Fehlings MG. Current status of experimental cell replacement approaches to spinal cord injury. Neurosurg Focus 2008;24:E19. Google Scholar | CrossrefPubMed | WorldCat
31) Groves AK, Barnett SC, Franklin RJ et al. Repair of demyelinated lesions by transplantation of purified O-2A progenitor cells. Nature 1993;362:453–455. Google Scholar | CrossrefPubMed | WorldCat
32) Rosenbluth J, Schiff R, Liang WL et al. Xenotransplantation of transgenic oligodendrocyte-lineage cells into spinal cord-injured adult rats. Experimental Neurology 1997;147:172–182. Google Scholar | CrossrefPubMed | WorldCat
33) Takami T, Oudega M, Bates ML et al. Schwann cell but not olfactory ensheathing glia transplants improve hindlimb locomotor performance in the moderately contused adult rat thoracic spinal cord. J Neurosci 2002;22:6670–6681. Google Scholar | CrossrefPubMed | WorldCat
34) Martin D, Robe P, Franzen R et al. Effects of Schwann cell transplantation in a contusion model of rat spinal cord injury. J Neurosci Res 1996;45:588–597. Google Scholar | CrossrefPubMed | WorldCat
35) Cummings BJ, Uchida N, Tamaki SJ et al. Human neural stem cells differentiate and promote locomotor recovery in spinal cord-injured mice. Proc Natl Acad Sci U S A 2005;102:14069–14074. Google Scholar | CrossrefPubMed | WorldCat
36) Karimi-Abdolrezaee S, Eftekharpour E, Wang J et al. Delayed transplantation of adult neural precursor cells promotes remyelination and functional neurological recovery after spinal cord injury. J Neurosci 2006;26:3377–3389. Google Scholar | CrossrefPubMed | WorldCat
37) Guest J, Herrera LP, Qian T. Rapid recovery of segmental neurological function in a tetraplegic patient following transplantation of fetal olfactory bulb-derived cells. Spinal Cord 2006;44:135–142. Google Scholar | CrossrefPubMed | WorldCat
38) Klussmann S, Martin-Villalba A. Molecular targets in spinal cord injury. J Mol Med 2005;83:657–671. Google Scholar | CrossrefPubMed | WorldCat
39) Totoiu MO, Keirstead HS. Spinal cord injury is accompanied by chronic progressive demyelination. J Comp Neurology 2005;486:373–383. Google Scholar | Crossref | WorldCat
40) Izrael M, Zhang P, Kaufman R et al. Human oligodendrocytes derived from embryonic stem cells: Effect of noggin on phenotypic differentiation in vitro and on myelination in vivo. Mol Cell Neurosci 2007;34:310–323. Google Scholar | CrossrefPubMed | WorldCat
41) Sharp J, Keirstead HS. Therapeutic applications of oligodendrocyte precursors derived from human embryonic stem cells. Curr Opin Biotechnol 2007;18:434–440. Google Scholar | CrossrefPubMed | WorldCat
42) Sheth RN, Manzano G, Li X et al. Transplantation of human bone marrow-derived stromal cells into the contused spinal cord of nude rats. J Neurosurg Spine 2008;8:153–162. Google Scholar | CrossrefPubMed | WorldCat
43) Hofstetter CP, Schwarz EJ, Hess D et al. Marrow stromal cells form guiding strands in the injured spinal cord and promote recovery. Proc Natl Acad Sci U S A 2002;99:2199–2204. Google Scholar | CrossrefPubMed | WorldCat
44) Franklin RJ, Gilson JM, Blakemore WF. Local recruitment of remyelinating cells in the repair of demyelination in the central nervous system. J Neurosci Res 1997;50:337–344. Google Scholar | CrossrefPubMed | WorldCat
45) Fernandes KJ, Fan DP, Tsui BJ et al. Influence of the axotomy to cell body distance in rat rubrospinal and spinal motoneurons: Differential regulation of GAP-43, tubulins, and neurofilament-M. J Comp Neurol 1999;414:495–510. Google Scholar | CrossrefPubMed | WorldCat
46) Hermann GE, Rogers RC, Bresnahan JC et al. Tumor necrosis factor-alpha induces cFOS and strongly potentiates glutamate-mediated cell death in the rat spinal cord. Neurobiol Dis 2001;8:590–599. Google Scholar | CrossrefPubMed | WorldCat
47) Nicholas R, Stevens S, Wing M et al. Oligodendroglial-derived stress signals recruit microglia in vitro. Neuroreport 2003;14:1001–1005. Google Scholar | PubMed | WorldCat